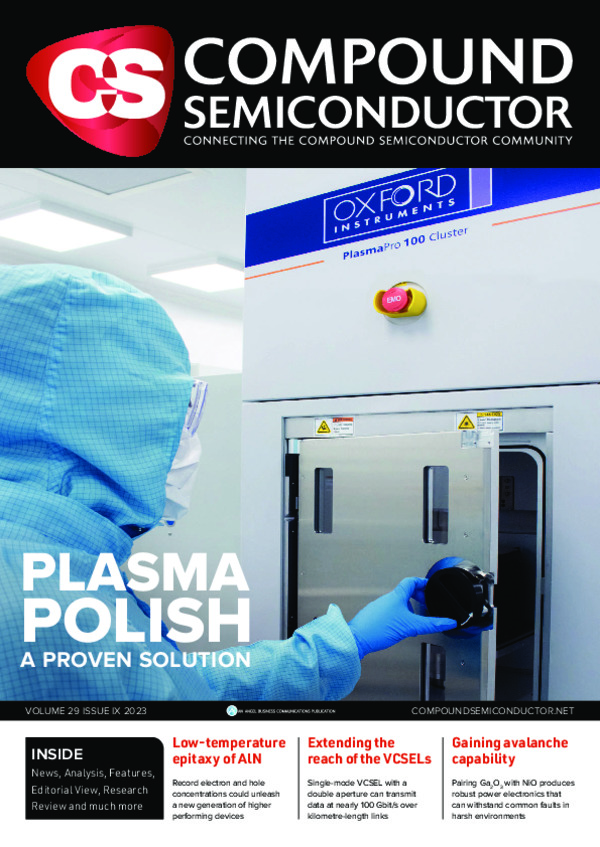
AlN: Opening doors with low-temperature epitaxy
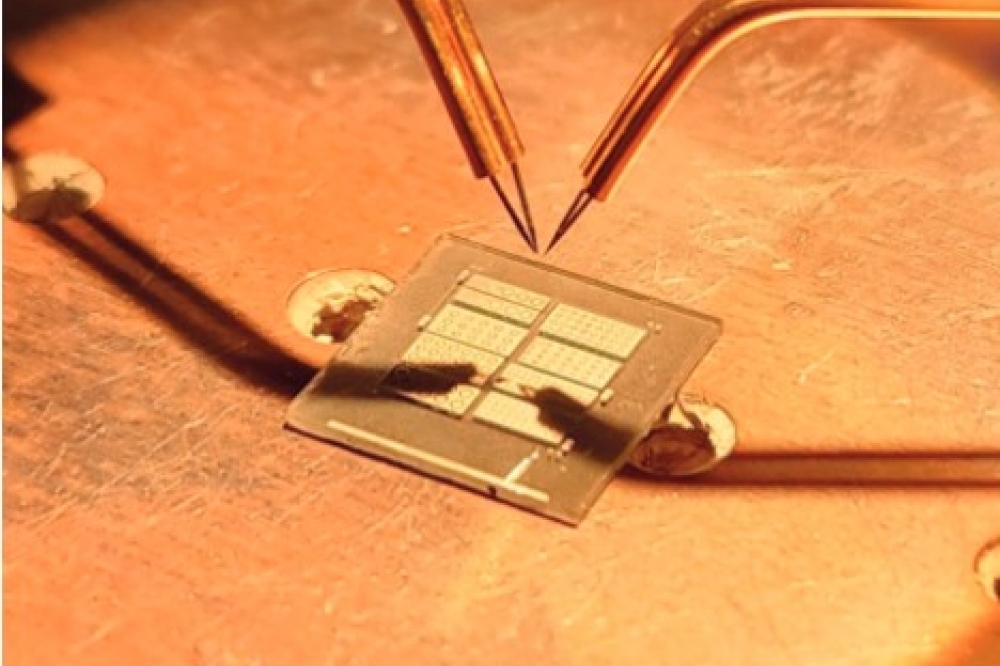
By delivering record electron and hole concentrations in AlN,
low-temperature epitaxy promises to unleash a new generation of extreme
bandgap devices.
BY CHRISTOPHER MATTHEWS, HABIB AHMAD, KEISUKE MOTOKI, SANGHO LEE, AHELI GHOSH, EMILY MARSHALL, AMANDA TANG AND W. ALAN DOOLITTLE FROM GEORGIA INSTITUTE OF TECHNOLOGY
Unfortunately today’s materials are not going to meet tomorrow’s needs. That’s not to say that GaN and SiC have not had substantial success – they are transforming power electronics and making solid-state lighting a reality. However, these wide bandgap materials have limitations when employed for high-power diodes and transistors for grid-scale electronics and electric vehicles, and they cannot be used to make ultraviolet emitters for sterilisation and lithography.
Offering much promise on all these fronts are a number of materials with even wider band gaps that have been attracting increasing attention in recent years. They include Ga2O3, diamond, and very recently, AlN.
For all of these materials, progress at the device level is far from easy. Ga2O3 has a number of great attributes, including a bandgap of 4.9 eV, a high critical electric field and compatibility with inexpensive bulk growth methods. But there are weaknesses: devices are limited to those that are unipolar, due to only n-type doping; and recent results have highlighted the low thermal conductivity of this oxide, hindering its applicability to power electronics.
Figure 1. Minimum required device thickness versus grid voltage for
various points along the power transmission grid, assuming the
theoretical critical electric field limits (taken from J. Y. Tsao et al.
Adv. Electron. Mater. 4 1600501 (2018)). Thicknesses below 200 µm are
considered reasonable for semiconductor devices. For higher voltage
transmission applications, every material requires an unreasonably high
device thickness. However, AlN is the only material compatible with
substation-level applications at both 230 kV and 138 kV. While multiple
materials can hold off lower voltages, AlN devices require the least
material, which makes them a comparatively economical choice.
Offering an even higher bandgap of 5.5 eV is diamond, which benefits from a high thermal conductivity and other favourable material properties. However, despite impressive recent substrate advances, diamond is difficult to dope and expensive to produce. This material is also hampered by its indirect band gap, making it unsuitable for optoelectronic applications, which have been a major leveraging factor for driving early investment and research in other semiconductors, such as GaN.
AlN has also garnered interest, thanks to its direct bandgap of 6.1 eV, its high critical electric field of 15.4 V/cm, its high thermal conductivity of 319 W/mK, and its compatibility with the established III-nitride infrastructure. However, doping AlN is challenging. Prior to 2020, reported carrier concentrations were limited to around 1010 cm-3 for holes and 1015 cm-3 for electrons.
Our team at Georgia Institute of Technology has smashed through this ceiling with metal-modulated epitaxy (MME), a low-temperature variant of MBE. Using MME, we have realised p- and n-type doping in AlN with beryllium and silicon dopants, respectively, obtaining carrier concentrations greatly exceeding previously reported values – they are well above 1018 cm-3. Read on to discover how low-temperature MME enabled this success.
Epitaxial considerations
In III-nitride semiconductors, the doping efficacy is largely influenced by the concentration of unintentional point defects. They include: contaminant atoms, typically oxygen, carbon, and hydrogen; cation vacancies; N-vacancies (VAl and VN for AlN); and reconfigurable defects, such as the DX-centre. Thus, it is of utmost importance to limit the incorporation of undesired impurities and the formation of vacancies, especially when trying to dope notoriously insulating materials, such as AlN.
These impurities, arising from various sources, are dependent on the growth technique. For oxygen and carbon contamination, two competing thermal mechanisms are at play. Higher temperatures increase the extent of the degassing of these elements from structural components within a growth chamber, but this is somewhat counterbalanced by a simultaneous decrease in their sticking coefficient on a semiconductor surface. To limit the incorporation of oxygen and carbon in thin films, growth is typically conducted at diametric temperatures – that is, as low or as high as possible.
For III-nitride epitaxy, the formation of vacancies is often thought to be related solely to the III/V (or V/III) ratio, with VAl occurring for N-rich growth and VN occurring for metal-rich growth, with the majority flux used to replace atoms lost to desorption. More generally, the concentration of vacancies is related exponentially to the temperature, so one useful strategy to reduce the vacancy concentration is to dramatically reduce the temperature.
One example of the diametric approach is MOCVD. This epitaxial growth technique employs high temperatures and thermodynamically produces high concentrations of VN. However, kinetically, the massive V/III ratio that’s used in MOCVD replaces these VN as they are produced.
The opposite approach, involving low temperature methods, never creates the enormous surface concentrations of vacancies by removing the thermodynamic driving force. Due to this, even excessive aluminium-rich III/V ratios lead to a low concentration of VN, regardless of the flux that’s used.
Figure 2. Representative calculations of the hoping rates and surface
diffusion lengths as a function of various atomic diffusion pathway
barriers. Nitrogen in a gallium bilayer has a diffusion length on the
order of one micron, even at low growth temperatures.
In most cases, the epitaxy of compound semiconductor layers is conducted near the highest temperature possible for that material, typically helping to promote the growth of smooth films. For the III-nitrides, this approach has been pursued to optimise films that are grown under nitrogen-rich growth conditions. However, there is a dramatic shift in physics for metal-rich growth that’s been overlooked. Essentially, high temperatures are needed in the nitrogen-rich regime to overcome large surface binding energy barriers and promote long-range adatom hopping, critical for the formation of smooth films. These barriers are much lower when adatoms exist on a metal-rich surface, ensuring that a similar degree of diffusion is possible at much lower temperatures.
It is easy to see these factors at play when comparing the diffusion lengths of the limiting reactants in GaN growth on different GaN surface terminations. For N-terminated (or dry) GaN surfaces, the diffusion barrier for gallium is between 1.4 eV and 1.8 eV. In comparison, the barrier is only 0.7 eV for nitrogen diffusion on a gallium terminated surface, and it is as low as just 0.12 eV for surfaces covered in a bilayer of gallium. Due to these variations, adatom surface diffusion lengths are significantly higher for metal-rich growth, exceeding 3 µm, compared with lengths for nitrogen-rich growth, even at much lower temperatures.
Figure 3. Typical flux profiles as a function of time in metal-modulated
epitaxy. Instantaneous metal flux (ΦIII) is higher than the nitrogen
flux (ΦN), ensuring that growth occurs in metal rich conditions. The
time-averaged metal flux (ΦIII,avg) is lower than (ΦN), eliminating
droplet build-up.
Based on this observation, reconsidering the use of high growth temperatures is worthwhile. After all, high quality III-nitride films can be realised at low growth temperatures using metal-rich conditions, while high growth temperatures have several downsides: there is an increase in impurity generation due to outgassing of structural components; greater thermal expansion takes place, introducing unwanted strain; and doping can be impaired.
Unfortunately, it’s not as easy as simply lowering the growth temperature and using exclusively metal-rich conditions. If that approach is adopted, it leads to an excess droplet build-up of metal, and ultimately has adverse effects on III-nitride film quality. What’s needed is a modulated growth technique, alternating between metal- and nitrogen-rich growth conditions. Typically implemented as a variation of MBE, this approach takes advantage of the benefits of metal-rich growth and limits the accumulation of excess metal.
Chief among these modified growth methods is MME. With this form of epitaxy, the MBE metal flux is in excess of the nitrogen flux by between 30 percent and 200 percent. A key part of the process is physically shuttering the metal sources, undertaken in a manner that ensures that the time-averaged growth conditions are nitrogen-rich through periodic removal of all metal accumulation (see Figure 3). When the metal shutters are opened, the surface is quickly terminated by a metal adlayer. Accumulation continues until the metal shutters are closed, at which point the metal adlayer is consumed by the continuous supply of active nitrogen. Once the metal adlayer is fully depleted, the film briefly stops growing until the metal shutters are re-opened and the cycle repeats. However, since the growth reaction only depends on the amount of adsorbed metal on the surface, rather than the metal flux, growth (almost) always occurs in metal-rich conditions.
Figure 4. Positions of and relative size (to scale) of magnesium, beryllium, and carbon acceptors in AlN.
Our group developed MME in 2007. Since then, we have used this form of epitaxy to deliver a number of impressive results in the III-nitrides, including: growth rates of nearly 10 μm/hr; single-phase ternary III-nitrides, without phase separation, throughout the miscibility gap of InGaN and at technically relevant compositions in the miscibility gap of AlInN; high n-type doping, with electron concentrations in GaN above 1020 cm-3; extremely high p-type doping, with hole concentrations in GaN above 6 × 1019 cm-3; and tunnel junctions in GaN with extremely low voltage drops (0.14 V), and in AlGaN alloys with up to 60 percent aluminium. Note that all this success has come without compromising film quality. Typical MME-grown films produce excellent X-ray diffraction figures-of-merit – that is, the rocking curve line widths match the substrate – alongside smooth surfaces, with a root-mean-square roughness below 1 nm, as has been demonstrated in multiple devices.
Initially our results, especially the doping successes, were fairly controversial, due to their incompatibility with the understanding of III-nitrides grown by traditional methods. For instance, prior theory and experimental results found that magnesium had a deep acceptor activation energy in GaN, limiting hole concentrations, but we found shallow acceptor activation in MME-grown films. This finding came as a surprise. Upon further inspection we realised that theoretical reports tend to only consider doping at levels close to the equilibrium solubility limit of the dopant impurities, a restriction that results in deep, isolated acceptor states in the band gap. With MME, growth is kinetically limited, allowing for deviations from equilibrium conditions, specifically hyper-doping beyond solubility limits. One consequence is that dopants can be forced into films at higher concentrations than the equilibrium solubility limit – and when the concentration is particularly high, the Bohr orbitals of these dopants start to overlap, creating a dopant band. For the magnesium dopant in GaN, formation of the acceptor band reduces the effective activation energies from around 210 meV to 50 meV. This leads to hole concentrations of nearly 1 × 1020 cm-3.
Following on from this work, we found an acceptor band in AlGaN with an aluminium composition of up to 60 percent. This observation provided the basis for investigating doped AlN.
Producing p-type…
We considered three potential dopants for p-type AlN: two of them were cation-substituted magnesium (MgAl) and beryllium (BeAl), and the other was anion-substituted carbon (CN). Magnesium is the natural first choice, due to its use as the primary p-type dopant for GaN. However, the highest reported hole concentrations in magnesium-doped AlN are less than 1010 cm-3. According to theoretical studies, magnesium behaves as a deep acceptor with an activation energy of 510-630 meV. While it is possible that this dopant could form a magnesium acceptor band, that’s unlikely. There is a reduced solubility of magnesium in AlN, coming from a large atomic radius mismatch between aluminium (118 pm) and magnesium (145 pm), as shown in Figure 4. It’s also worth noting that there are reports of a reduced efficacy of magnesium-doping on hole generation as the aluminium-content in AlGaN is increased, and thus, the average cation size is decreased.
Figure 5. Temperature dependent Hall measurements of p-AlN showing an
activation energy of 37 meV. Jeff Lindemuth from Lake Shore Cryotronics,
Inc. is thanked for performing this measurement.
Carbon is a similarly unrealistic dopant, but for different reasons. While there is a report of limited, surface-level p-type conduction in carbon-doped AlN, this candidate for doping is yet to provide substantial bulk hole concentrations in AlN and it is commonly understood to be a compensating impurity in AlN. Another major concern is that the carbon concentrations in MOCVD-grown III-nitrides are inconsistent from run-to-run, due to carbon in the metal-organic sources. The likelihood is that this unwanted variation would make carbon doping difficult to control.
That leaves beryllium as the most promising p-type dopant. While it’s also predicted to be a deep acceptor, having an activation energy of 220-340 meV, it does not suffer from the same issues as carbon and magnesium. Unlike magnesium, there’s a fairly small atomic radius mismatch, because the radius for beryllium is 112 pm (see Figure 4). Due to this, there is the promise that the high solubility of beryllium in AlN enables acceptor band formation. Our recent studies support this possibility, with investigations determining a room-temperature hole concentration of 4.4 x 1018 cm-3 in beryllium-doped AlN, along with an effective activation energy of 37 meV (see Figure 5).
Based on work from the Nobel Prize-winning condensed matter physicist Sir Neville Mott, in partnership with W.D. Twose, it is possible to estimate the critical dopant concentration at which an energy band begins to form. For beryllium in AlN, the beryllium concentration in AlN could be predicted to be a staggering 4 x 1021 cm-3, equating to roughly 4 percent of the atomic density of AlN.
Why so high a value? It’s because the calculation assumes that the valence band structure of AlN is similar to other III-nitrides – and that’s not actually the case. For AlN, the split-off band has greater curvature and is closer in energy to the conduction band than the heavy- and light-hole bands. Due to this, the lowest-energy holes in AlN have a much lower effective mass than those in the other III-nitrides. Once this is accounted for, the critical dopant concentration for acceptor band formation is amended to just 1.2 x 1018 cm-3. It’s a value that agrees with experimental evidence, which indicates that p-type conduction is only measurable for beryllium concentrations beyond the high 1017 cm-3 range, where sufficient holes exist to allow respectable contacts.
Despite our successes, there are still challenges with beryllium-doping in AlN, primarily associated with the growth temperature. Our biggest temperature-dependent concern is that beryllium has an intermediate vapour pressure, so it is very easily desorbed, even at relatively low growth temperatures of just over 750 °C. Due to this, growing beryllium-doped AlN at high temperatures results in reduced beryllium incorporation and thus a reduced hole concentration. Cranking up the beryllium flux is not an option, as too much beryllium desorption in the growth chamber can result in beryllium runaway, making the chamber itself an uncontrollable source of beryllium.
Another important consideration when doping AlN with beryllium is to limit the number of compensating defects that may arise in the film, such as VN and oxygen – the latter is particularly attracted to aluminium and beryllium, and may form ON and Be-O defect complexes. As discussed previously, it is possible to limit VN defects by appropriately tuning MME growth conditions, and to reduce the generation rate of oxygen by growing at low temperatures where metal components do not outgas.
One other issue that arises with beryllium is that due to its small size – it is slightly smaller than the aluminium cations – it can physically reconfigure in the lattice, transforming from a BeAl acceptor to a compensating beryllium interstitial. As this reconfiguration is more likely to occur in films grown at high temperatures, due to thermal expansion, this is yet another reason why it is vital to grow p-type AlN at low temperatures.
… and n-type material
For n-type AlN, potential dopants are anion-substituted oxygen (ON) and cation-substituted silicon (SiAl), as shown in Figure 6. However, we can quickly rule out oxygen, as it only generates shallow donors in AlN when it is combined with significant aluminium-vacancy concentrations to form negatively-charged defect complexes, and that limits its usefulness.
Fortunately, silicon doping has been slightly more straightforward, enabling bulk electron concentrations of 6 × 1018 cm-3, which represents nearly a 3000-fold improvement over the previous state of the art. While silicon has a much lower vapour pressure than beryllium, and thus will not desorb at typical III-nitride growth temperatures, it appears that the low growth temperatures for MME still play a crucial role, with silicon doping benefiting from low temperature, metal-rich growth conditions.
The major complication associated with silicon doping of AlN is the silicon-DX centre, which forms during the breaking of the c-axis bond between a SiAl atom and its neighbouring nitrogen atom. Like with beryllium doping, higher growth temperatures are undesirable, as they can result is greater thermal expansion and promote an undesirable lattice reconfiguration. Furthermore, the presence of VAl defects can ‘soften’ the lattice, making it easier for these types of DX-centres to form. To combat this, the approach we have already discussed – the use of metal-rich growth – can dramatically lower the likelihood of VAl formation.
So far, the most common approach to trying to dope AlN has been via MOCVD, which requires high growth temperatures and nitrogen-rich growth conditions. Both are impediments to realising a high efficacy for silicon doping. An additional impediment is the compensating nature of carbon, which is inevitable in MOCVD-grown films. With all these factors at play, maybe it’s not surprising that we have been able to exceed the previous state of the art in n-type AlN by such a large margin.
Figure 6. Positions of and relative size (to scale) of silicon and
oxygen donors in AlN, as well as the compensating silicon DX-centre
configurations.
Developing devices
Armed with the capability to produce reasonably high hole and electron concentrations in AlN by MME, we have demonstrated rectification in a p-i-n diode. Our preliminary diodes show six orders of magnitude of rectification, an ideal turn on voltage at nearly 6 V, and a current density of up to 2.8 A cm-2.
The performance of these devices is dominated by a high series resistance in forward bias, probably originating from contact issues to the etched n-type surface of these quasi-vertical diodes that need to be addressed. Our initial theories for the high contact resistance include a fundamental issue when contacting a 6.1 eV band gap material with metals whose work functions are limited to 2.8 eV to 5.8 eV, and damage to the n-AlN film from non-optimised plasma etching.
We have carried out temperature-dependent current-voltage characterisation of these diodes, which revealed a thermally activated process at voltages above turn-on. For voltages below turn-on, the current is independent of temperature, suggesting contacts are dominated by tunnelling rather than traditional ohmic conduction. We need to undertake further investigation of our diodes, so that we can determine the physical processes that dictate the electrical characteristics of AlN diodes. Such efforts must include investigations to establish better contact solutions, which will remove one complication when analysing the current-voltage curves and enable higher current devices.
There is no doubt that low-temperature epitaxy plays a vital role in realising highly p- and n-doped AlN and AlN diodes. The low temperatures allow beryllium and silicon dopants to incorporate into AlN films without reconfiguring into compensating centres. This limits the creation of compensating vacancies in AlN, and reduces the levels of compensating impurities, such as carbon and oxygen, that outgas from structural components in a growth chamber.
Thanks to the large diffusion lengths of adatoms on metal-rich surfaces, metal-rich vacuum processes can be conducted at these low temperatures without sacrificing the structural quality of these films. By avoiding the complications that arise from dopant desorption, beryllium incorporates into AlN films at high enough concentrations to form an acceptor band, greatly reducing the effective activation energy and improving the viability of p-type AlN. Another benefit of this material combination is that due to the unique valence band structure for AlN, the impurity band forms at much lower beryllium concentrations than in GaN, giving rise to the promise of much higher hole mobilities.
Measurements on our MME-grown films of p- and n-type AlN reveal carrier concentrations of 4.4 x 1018 cm-3 and 6 x 1018 cm-3 and resistivities of 0.045 Ω cm and 0.02 Ω cm, respectively. We have also recorded a hole mobility for AlN that is ten times higher than that for GaN at the same carrier concentration, suggesting AlN may offer more promise for p-type devices. While much work is still needed, there are encouraging signs that AlN offers the solution to the search for a material that will handle future performance needs in electronics and optoelectronics.