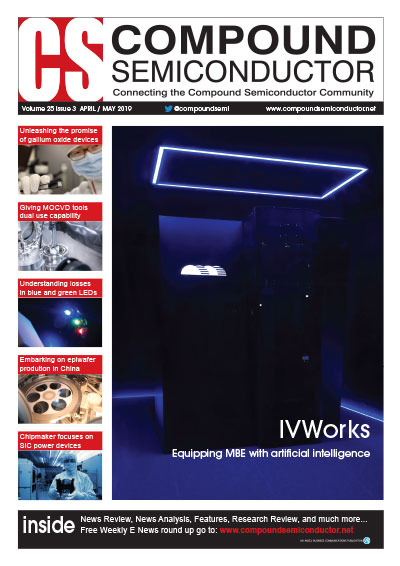
Understanding inefficiencies in blue and green LEDs
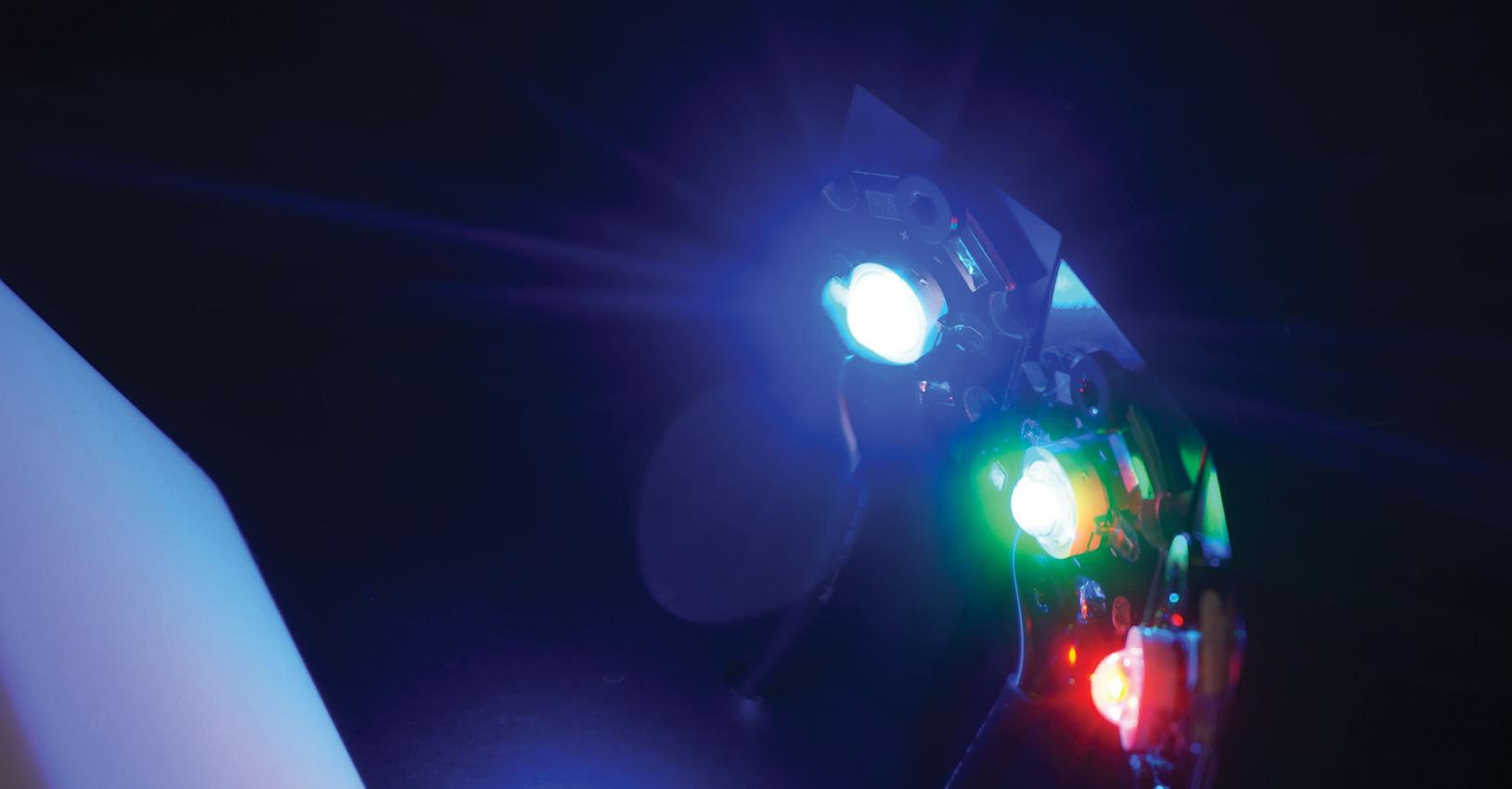
The three major weaknesses of GaN LEDs – the efficiency droop, the green gap, and the electrical potential drop – all result from changes to the recombination coefficients, which vary with carrier density
BY DONG-PYO HAN, SATOSHI KAMIYAMA, TETSUYA TAKEUCHI, MOTOAKI IWAYA AND ISAMU AKASAKI FROM MEIJO UNIVERSITY AND NAGOYA UNIVERSITY, JAPAN
What is the perfect source of light? Surely, it is one that waste no energy in producing photons that span the deepest violets to the near infra-red, much like the visible rays from the sun. Judged in these terms, the emission from solid-state sources that combine the output of red, green and blue LEDs is encouraging. However, there is still much room for improvement.
Consider the blue and green sources, made from nitride-based LEDs that feature GaInN quantum wells. For these devices, performance is pegged back by three phenomena: droop, the gradual decrease in efficiency as the drive current increases; the green gap, the gradual decrease in efficiency as the indium content in the multiple-quantum-wells rises, to push emission to longer wavelengths; and the electrical potential drop, which leads to dramatic increases in the voltage that must be applied to provide an increase in the driving current.
Addressing all three of these would lead to a dramatic improvement in the quality of the LED light source. It would allow it to operate at a higher efficiency, while delivering a strong spectral output across the entire visible spectrum.
Much effort has already been devoted to uncovering the physical mechanisms responsible for the efficiency droop and the green gap. Researchers have considered carrier overflow/spill-over, carrier localization/delocalization, piezo electric fields, and asymmetric carrier distributions in multiple quantum wells, and tunnelling leakage currents. Many of these investigations have concluded that an increase in a non-radiative recombination process is to blame for the declines in efficiency with higher current densities and longer wavelengths.
Meanwhile, the numerous studies on the electrical potential drop have tended to focus on either current crowding caused by a lateral structure, an insufficient contact potential barrier between the metal and the semiconductor, a high magnesium-acceptor activation energy, or a heavy effective mass for the hole. Often, the problem has been thought to be due to the ohmic potential drop.
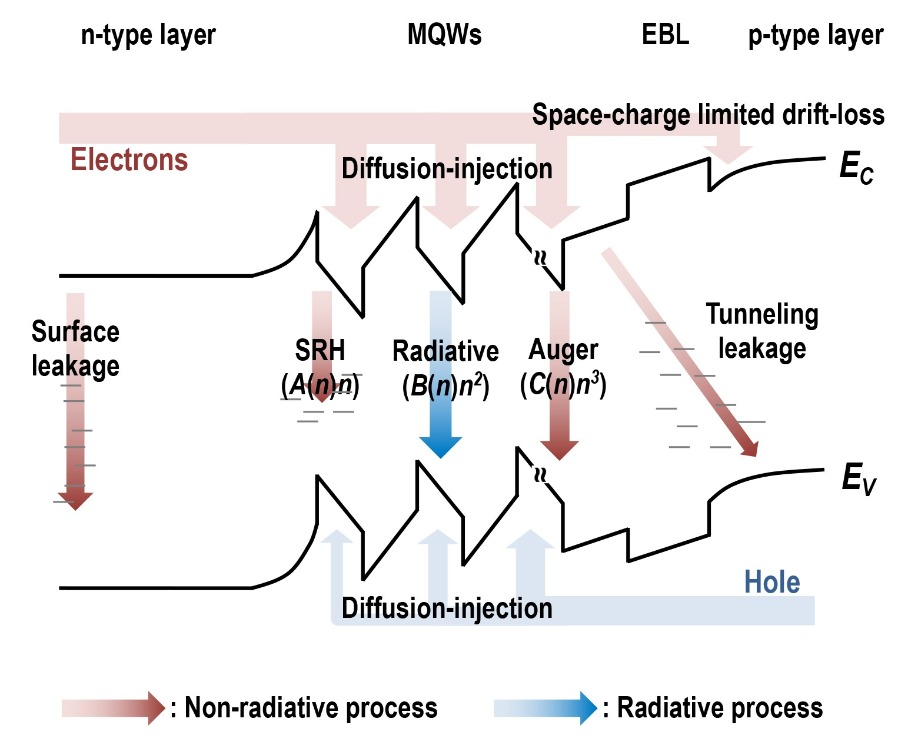
Figure 1. Overall carrier transport/recombination process in the operation of the GaInN-based LED.
Our team at Akasaki laboratory, Meijo University is taking a different approach to trying to understand the limitations of GaN-based LEDs. We are less concerned with an explanation for droop, potential drop, and the green gap, and have instead set ourselves the goal of understanding the recombination and transport process inside and outside multiple quantum wells, and how this relates to the inefficiencies withiin the LED.It is well-established that a lower value for the internal quantum efficiency occurs when fewer electrons that are injected into the quantum well are converted into photons. When the internal quantum efficiency falls, this can account for the green gap and the efficiency droop – and it can also be an indirect cause for the electrical potential drop. So, it is clearly important to understand the internal quantum efficiency of the LED, as this can aid the development of artificial white-light sources that take efficiency to a new level.
We have developed an accurate understanding of the internal quantum efficiency of the LED by beginning with an examination of the carrier transport and recombination processes inside the device, using an energy-band diagram (see Figure 1). There are essentially three parts to carrier transport: diffusion-injection from n- and p-bulk layers into the multi-quantum well active region; space-charge limited drift-loss to the opposite bulk layer, due to carrier overflow or spill-over; and leakage currents, arising through the surface defect and the tunnelling leakage current through threading dislocations.
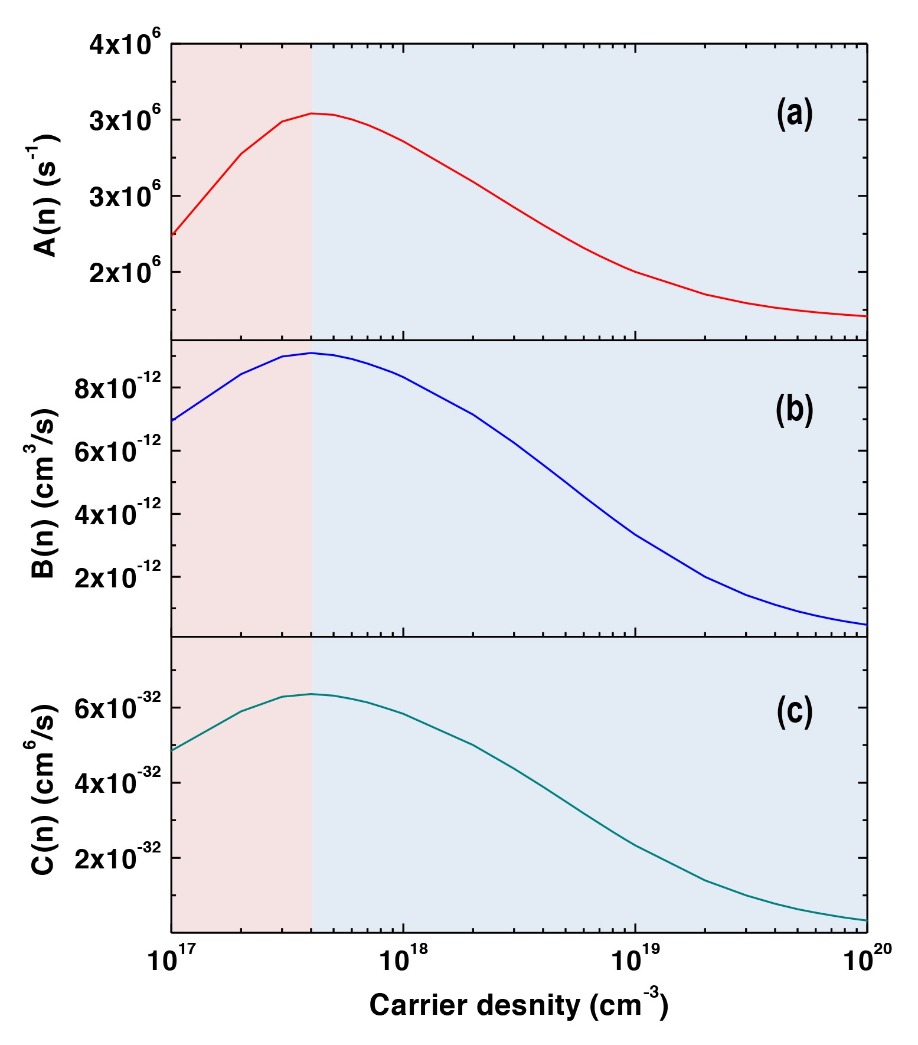
Figure 2. Numerical calculation results of (a) A(n), (b) B(n), and (c) C(n) coefficients as a function of carrier density n, respectively.
Of these three, the only carrier transport process that has the possibility of leading to light generation is that of diffusion-injection into the multiple quantum wells. Once carriers are in this active region, they may either undergo the non-radiative, heat-generating processes of Shockley-Read-Hall recombination via an internal defect and Auger recombination, or they may emit light via bimolecular recombination. So, in short, the only process that leads to light generation is diffusion-injection into the multiple quantum wells, followed by bimolecular recombination.To arrive at a deep understanding of the internal quantum efficiency, it is essential to examine the coefficient of each recombination process. As shown in Figure 1, the carriers that are inserted into the multiple quantum wells from the n-type and p-type layers lose their energy through each recombination process. In general, up until now, simply A, B, and C coefficients have been used to express the various processes in the form of the terms, An, Bn2, and Cn3, that are united in what is known in the LED research community as the ABC model. Within this framework, the A, B, and C coefficients are considered to be fixed in value, and independent of the carrier density.
Varying coefficients
However, our research reveals that this approach is fundamentally flawed. That’s because the ABC coefficients are not fixed, but have great dependency on the drive current and the carrier density (see Figure 2). For all three coefficients, values rise with carrier density, before peaking and then declining substantially.
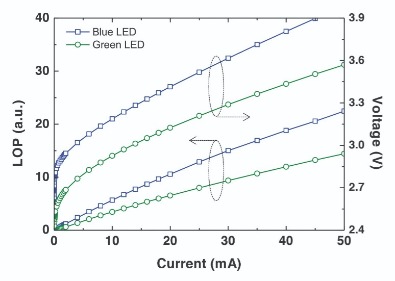
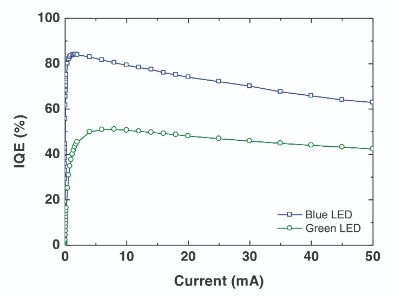
Figure 3. Measurements of (a) the light output power and applied voltage as a function of driving current and (b) the internal quantum efficiencies (IQEs) as a function of driving current for the blue LED and green LED, respectively.
We attribute the rising value of the ABC coefficients to the quantum-confined stark effect and the carrier screening effect. Behind the subsequent fall is the high-level-injection in A, and phase-space filling in B and C. Ignore this and treat the ABC coefficients as constant, and there is the danger of failing to understand the actual phenomena within the LED.To gain a greater insight into the two efficiency degrading issues, it is critical to be able to measure the internal quantum efficiency of the LED as a function of drive current. Recently, we have developed a highly reliable way to do this, deriving a value from dependency of the general ABC coefficients.
Our approach is to determine the absolute value for the internal quantum efficiency from one of the properties of the ABC coefficients – that they have inflection points at the same carrier density. We also draw on the mathematical model, making use that the differential of the radiative current and non-radiative current is constant only at the driving current where ABC coefficients have inflection points.
Using this approach, our measurements on blue and green LEDs that were made by our collaborators at Meijo University have enabled us to produce plots of light-output-power as a function of driving current and applied voltage (see Figure 3 (a)). As expected, the light-output-power of the green LED is generally lower than that of the blue LED. Also note that at high currents, the electrical potential drop – that is, the slope of the current-voltage curve – is higher for the blue LED than its green cousin.
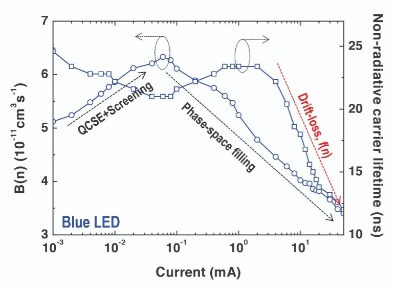
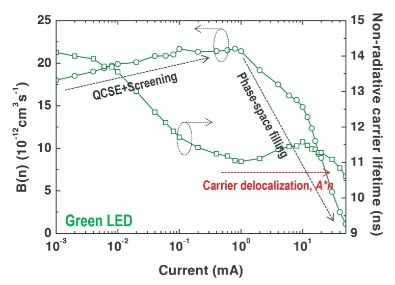
Figure 4. Measurement results of B(n) and non-radiative carrier lifetime as a function of driving current for the (a) blue LED and (b) green LED, respectively.
Using our approach, we have also plotted the internal quantum efficiency as a function of drive current. As expected, these graphs illustrate two of the biggest weaknesses of GaN LEDs, namely efficiency droop and the green gap. It is also evident that the drop in internal quantum efficiency is behind the lower light-output-power of the green LED, compared with its blue cousin. Clearly, the goal for the LED community is to develop a device that has a small electrical potential drop, alongside an internal quantum efficiency that is high, even at high drive currents and longer wavelengths.To examine the three efficiency degrading issues in more detail, we have delved deeper into the LED’s carrier recombination and transport dynamics. While doing so, we have made sure that we have investigated the recombination coefficients as a function of current for each sample.
Recently, we reported that one way to determine the recombination coefficient as a function of drive current is to combine the results of the how the: differential carrier lifetime, which is determined from impedance measurements, varies with drive current; with the result of plots of the internal quantum efficiency for different currents. Using this method, we have measured the recombination coefficients as a function of current for blue and green LEDs (see Figure 4(a) and (b)).
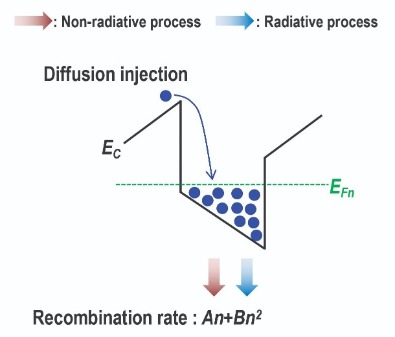
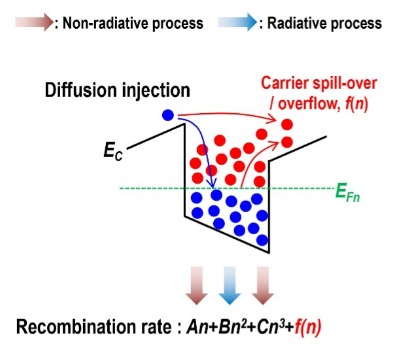
Figure 5. Carrier dynamics inside the multiple quantum well (MQW) of a blue LED: (a) at low current density; (b) at high current density.
Our measurements show that for both the blue and the green LED, the B coefficient, which is dependent on the carrier density, varies with current in the manner predicted by theory. That is, the value for B initially rises with current, before continuously falling after it peaks. Note, however, that there are differences between theory and measurement in the value of B, and the rate of its change.Comparing blues and greens
Comparing the blue and green devices reveals that the value of B for the green LED is about ten times smaller than that of its blue sibling, and its degree of decrease at high current is more severe. However, for the blue LED this coefficient decreases rapidly in the non-radiative carrier lifetime high-current region, while the green emitter does not exhibit such a drastic change. Note that for both the blue and green LEDs, the absolute value of non-radiative carrier lifetime at high-current region are similar to each other. That is, the non-radiative process is not the primary cause of the green gap.
Armed with these insights, we have searched for the reason behind the similarities and the differences in the carrier recombination and transport characteristics of the green and blue LEDs. We were also keen to discover how these characteristics would impact LED performance, and what their relationship is with the aforementioned three efficiency degrading phenomena.
Our efforts have given us a new insight into the behaviour of both blue and green LEDs. For blue LEDs, when driven at a low current, the carrier recombination rate is considerably larger than the carrier injection rate. In this regime, every injected carrier recombines, losing its energy inside the multi-quantum well, which has a carrier density determined by the quasi-Fermi level (see Figure 5(a)).
As the current is cranked up, the ABC coefficients gradually decrease, and the increase in the recombination rate fails to keep pace with the increase in the injection rate. Consequently, there comes a time when the carrier injection rate exceeds that of the carrier recombination rate.
At this point, carriers start to gradually accumulate inside the multi-quantum well. In some cases, they even show the same characteristics as those of the hot carrier. If they do, they acquire enough energy to overcome the barrier and escape from the wells to the opposite layer. This spill-over, or overflow, results in efficiency droop (see Figure 5(b)). For the hot carriers that accumulate inside the wells, the result is a space-charge, with current flowing in the form of a space-charge limited drift-leakage current that causes the electrical potential drop (see Figure 1 and Figure 3(a)). So, the efficiency droop and the electrical potential drop are interconnected phenomena in blue LEDs, both caused by a decrease in the recombination coefficient.
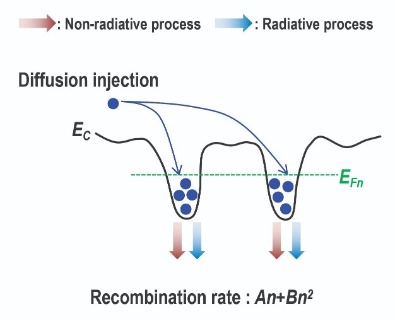
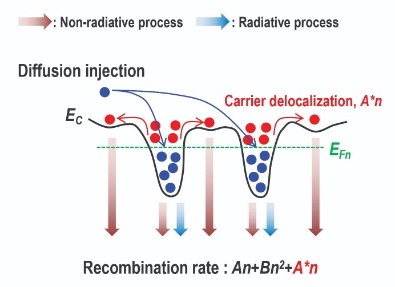
Figure 6. Carrier dynamics inside the multiple quantum well (MQW) of a green LED: (a) at low current density; (b) at high current density.
The behaviour of the green LED differs from that of the blue, due to the high indium content inside the multi-quantum well that causes alloy fluctuations, resulting in potential fluctuation and carrier localization. When this type of device operates at a low drive current, carriers do not stray far from the low potential area. Operating in this regime, the effective recombination volume of the green LED is smaller than that of its blue cousin (see Figure 6(a)).Like the blue LED, the ABC coefficients of the green LED decrease gradually with increases in current. Due to this, there is a point at which the carrier injection rate of the green LED exceeds the carrier recombination rate, and the accumulated carriers exit from the localization centre. However, the key difference with green LEDs is that the carriers that exit the localization centre lose their energy in additional Shockley-Read-Hall recombination when inside the wells, due to the high density of non-radiative recombination centres inside this active region.
Judged in terms of the non-radiative carrier lifetime, there is just a small difference between the blue LED and the green LED (see Figure 4). But the value for the B coefficient, which is a function of the carrier density, is about ten times smaller for the green LED than itsblue cousin. This implies that the difference in internal quantum efficiency (see Figure 3) – that is the green gap – is mainly determined by the difference in the B coefficient.
Why should the B coefficient vary so much between the blue and green LEDs? It is due to the complex interaction between: the quantum-confined Stark effect, which is caused by the relatively strong piezo electric field; and the decrease in the density of state of active region, resulting from the potential fluctuation followed by the decrease in the quantization dimension for the green LED. The quantum-confined Stark effect drives apart the hole and electron wave-functions in real-space, while the localization results in the separation of the hole and the electron wave-functions in k-space. The upshot is a dramatic fall in the carrier-density-dependant B coefficient, which accounts for the green gap.
Our work shows that efficiency droop is caused by the decrease of the ABC coefficient, triggering space-charge limited drift-loss that makes an electrical potential drop, while the green gap is caused by the decrease of B itself. While the non-radiative processes caused by them are different, the cause of both these phenomena is the same: the degradation of the B coefficient.
We have studied the three biggest impediments to high-efficiency LEDs: the efficiency droop, the green gap, and the electrical potential drop. Our work breaks new ground by examining all three of these maladies from the viewpoint of the reduction of recombination inside the multi-quantum well. All three impediments are, in principle, identical. Therefore, they can be simultaneously addressed by improving the radiative recombination among the recombination processes within the wells.
The authors would like to thank Prof. Dong-Soo Shin and Prof. Jong-In Shim at Hanyang University, Korea, for the valuable discussion and motivation. This work was supported by MEXT Private University Research Branding Project, MEXT Program for research and development of next-generation semiconductor to realize an energy-saving society, JSPS KAKENHI for Scientific Research A [No.15H02019], JSPS KAKENHI for Scientific Research A [No.17H01055], JSPS KAKENHI for Innovative Areas [No.16H06416] and Japan Science and Technology CREST [No.16815710].
Further reading
D.-P. Han et al. Phys. Status Solidi A 210 2204 (2013)
D.-P. Han et al. Appl. Phys. Lett. 105 191114 (2014)
D.-P. Han et al. Appl. Phys. Express 9 081002 (2016)
D.-P. Han et al. Appl. Phys. Express 10 052101 (2017)
D.-P. Han et al. Appl. Phys. Express 10 122101 (2017)
D.-P. Han et al. IEEE J. Quantum Electron. 54 3200107 (2018)
D.-P. Han et al. Appl. Phys. Express 12 032006 (2019)