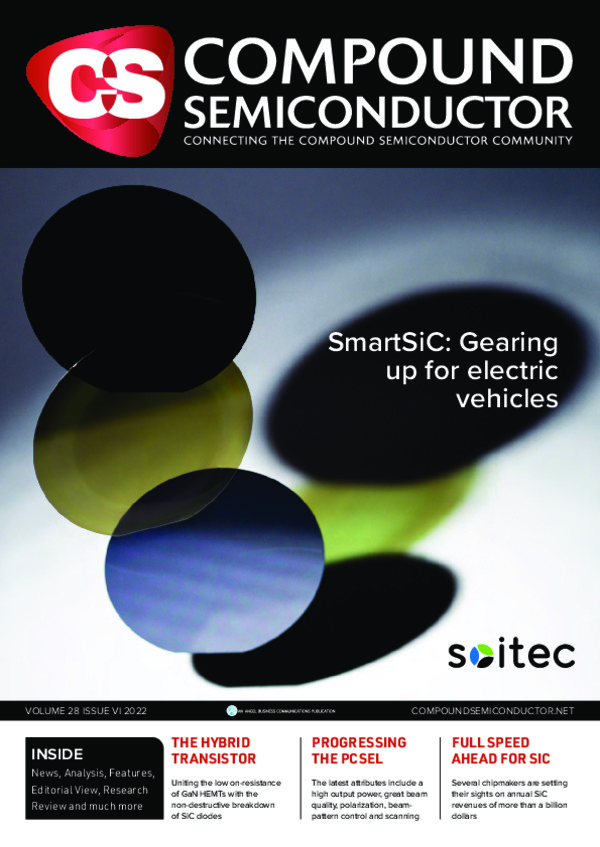
Advancing InGaN epitaxy for long-wavelength LEDs
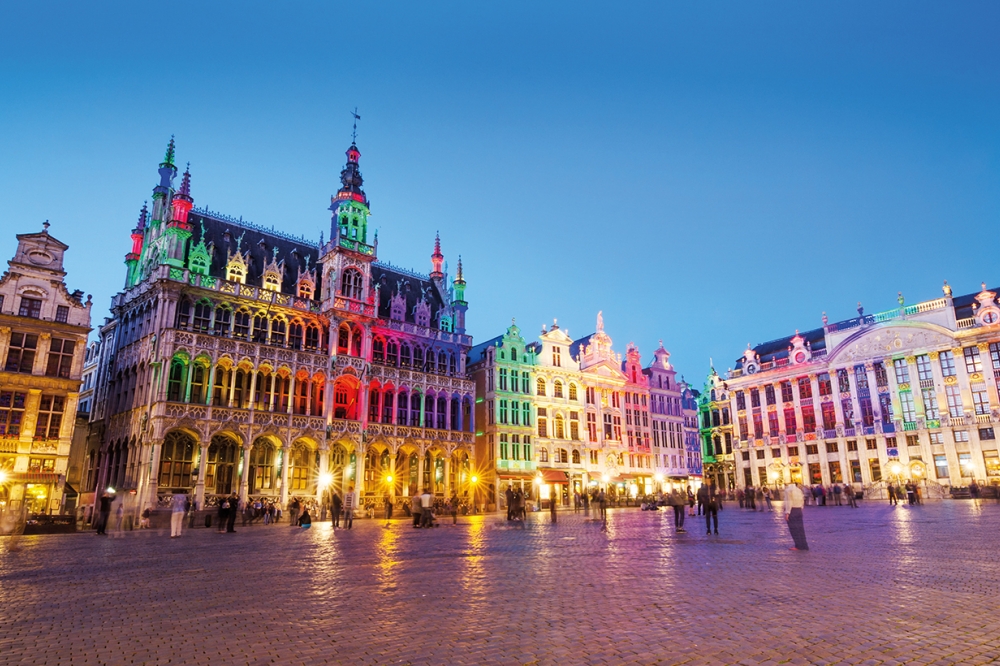
Indium-rich InGaN, the established material of choice for green LEDs, is
now starting to challenge traditional red-emitting materials in
microLEDs for head-mounted displays.
BY ROB ARMITAGE, TSUTOMU ISHIKAWA, TED CHUNG AND ZHONGMIN REN FROM LUMILEDS
The age of LED lighting is now upon us. Today white LEDs, utilizing phosphor-converted InGaN blue pump sources, are ubiquitous in general illumination. Due to this, attention has shifted away from blue LED technology, with the focus now on improving InGaN epitaxy at longer wavelengths, as this holds the key to more efficient emission in this spectral domain. Such efforts are motivated by a desire to increase the wall plug efficiency (WPE) of green and amber LEDs, and ultimately improve phosphor-free illumination systems based on colour mixing. This approach has already gained some traction at current system-efficacy levels, thanks to the benefits that colour-tuneable lighting brings to human health and well-being. In future, there is the tantalising prospect of building next-generation systems that exceed the efficacy of phosphor-converted blue light sources, enabled by large improvements to the WPE of LEDs in the green-amber range. Getting there, though, will not be easy.
When increasing the emission wavelength of the InGaN LED, there is never an abrupt decline in the internal quantum efficiency (IQE). Instead, it gradually decreases – although this adds up to a large difference between the external quantum efficiency (EQE) of state-of-the-art blue and green emitters (see Figure 1). Inspect the plots of the EQE at various currents, and it is clear to see that the wavelength dependence of InGaN efficiency is intertwined with that of the widely discussed malady known as ‘droop’. These phenomena are naturally interconnected, given that they are governed by fundamental recombination physics and non-radiative Auger recombination.
Figure 1. The external quantum efficiency of state-of-the-art visible
wavelength LEDs with 1 mm by 1 mm dimensions and a hemispherical dome
encapsulation.
Another factor at play in InGaN LEDs, which explains many of its unusual characteristics, is the crystal polarization charges that exist at the interfaces between the quantum wells and barriers. Present in conventional devices formed by epitaxial growth along the c-axis orientation, these charges give rise to internal electric fields across the QWs – and as the indium content in these wells increases, the quantum-confined Stark effect (QCSE) get stronger.
It’s worth noting that the strong QCSE in InGaN LEDs is actually a blessing and a curse. Its benefit is that by shifting both the electron and the hole energies, it is possible to realise green and even red emission with QW indium concentrations that are manageably low in terms of the challenges of crystal growth. But this blessing comes at the cost of a comparatively long radiative recombination lifetime, of the order of 100 ns. Due to this, degenerate hole densities arise in green LEDs at current densities below 10 A cm-2, and the Auger process becomes the primary recombination mechanism at practical operating conditions. The higher carrier densities reached in longer wavelength InGaN LEDs impair performance, increasing the severity of IQE droop, inducing a blue shift, and broadening the emission spectra at higher drive currents. Working in tandem, these drawbacks are so significant that they are holding back the large-scale deployment of green and yellow LEDs in solid-state lighting, especially for applications requiring high luminance.
To address all these technical challenges, our team at Lumileds has been participating in a project funded by the US Department of Energy that is trying to increase the efficiency of InGaN LEDs at longer wavelengths. Working with us are collaborators from the University of Michigan, the University of New Mexico, Ohio State University and Sandia National Laboratory.
Our strategy for realising far-more-efficient green LEDs is to engineer the epitaxy so that we utilise many QWs, thereby reducing the average carrier density within the active region. While this may appear to simply involve increasing the number of QWs, it’s actually more subtle. Success hinges on evenly distributing electrons and holes across multiple quantum wells – a goal that is frustrated by an imbalance of the respective carrier mobilities, as well as energy barriers to carrier injection and inter-well transport, associated with interface polarization charges.
To enjoy success, we exploit ever-present threading dislocations that arise from GaN epitaxy on substrates such as sapphire and silicon. By controlling growth conditions, we open or close pits around dislocations, as this allows us to produce devices that are three-dimensional at the microscopic scale and enhance hole injection through the lateral direction. With this strategy we have fabricated LEDs that have an EQE exceeding 40 percent and a dominant wavelength of 525 nm when driven at a current density of 35 A cm-2. As well as diminished droop (see Figure 2), these optimized green multiple-QW designs offer reduced sensitivity of spectral characteristics to changes in current density (see Figure 3).
These observations are consistent with our understanding that non-radiative recombination and shifts in spectral properties have a common origin, namely a high carrier concentration, and that both these weaknesses can be addressed with LED designs that drive down the average carrier density. Note that even for our green LEDs, specifically optimized for high-power operation, employing a lower current density ensures large efficiency gains (see Figure 2).
Figure 2. External quantum efficiency as a function of current density
for optimized green LEDs with various numbers of quantum wells in the
active region.
From green to red
For wavelengths longer than 590 nm, today’s AlInGaP LEDs have a much higher intrinsic efficiency than their InGaN cousins (see Figure 1). While this is likely to always be the case, the emergence of microLED display technology has generated considerable interest in InGaN red epitaxy. Partly fuelling this interest is a comparison between the respective efficiency levels for different colours – this identifies that regardless of the material system, the red LED consumes the majority of the power in a display.
There are several arguments in favour of a switch from red AlInGaP LEDs to those made from InGaN. Moving to InGaN for the red content would simplify the complexity of integration, as all sub-pixels would be based on the same materials system. Attempts to realise monolithic integration would also face fewer hurdles. In some cases, these gains could be so compelling that they could counter the hit to efficiency. Another consideration supporting a switch to InGaN is that despite its lower intrinsic efficiency, it might outperform AlInGaP at sufficiently small pixel sizes, due to its slower surface recombination velocity. Whether that’s actually the case is an important question with an ever-changing answer, influenced by improvements to epitaxy and die passivation technologies.
We are continuing to consider this matter, updating our view as we advance both materials systems. For InGaN, the process that we use to make red epitaxial wafers employs standard LED production substrates and reactors. By taking this approach, our InGaN wafers for blue, green and red LEDs are interchangeable in die fabrication. This contrasts with many exotic approaches for making red LEDs, such as selective-area epitaxy, porous GaN templates and InGaN pseudo-substrates. All are potentially interesting from a performance standpoint, but come with obvious disadvantages in manufacturing cost and complexity.
Figure 3. Spectral parameters versus current density for green LEDs
shown in Figure 2. Dashed lines indicate the full-width at half maximum
(FWHM) and solid lines indicate the peak wavelength shift relative to
its value at a low current density.
The emission of a representative InGaN red LED produced by our team is broad, but spatially homogenous at the microscopic scale, even for a very low injection current (see Figure 4). For such broad spectra the dominant wavelength is substantially shorter than the peak wavelength; due to this, in order for the eye to actually perceive this emission as red, the peak wavelength must sit well within the red. While InGaN LEDs with peak wavelengths as short as 600 nm have been referred to as ‘red’ in the literature, they actually fail to meet the minimum colour requirements for display applications. Our epitaxy is capable of producing LEDs with true red emission at current densities up to 50 A cm-2. These devices have wall-plug efficiencies comparing favourably with those published for InGaN LEDs of similar dominant wavelengths.
Figure 4. Spectrum of an InGaN red LED operated at 40 A cm-2 with a peak
wavelength of 638 nm and a dominant wavelength of 610 nm. The inset
shows the emitting surface observed under an optical microscope at the
forward voltage of 1.8 V, corresponding to the onset of light emission.
One of the issues of a shift in wavelength with drive current is that it could lead to variations in the colours produced by a display at different brightness levels. While this is easy to overcome with pulse width modulation (PWM), the sensitivity of the InGaN red spectrum to the current density has implications for display applications. When deploying blue and green LEDs in displays, it is common practice to either plot the efficiency as a function of current density for one device, or for a group of devices with the same wavelength target (see Figure 5). But for InGaN red LEDs, a better way to visualize the accompanying data is to use a contour plot that captures the characteristics of many LEDs, with differences in wavelength obtained by controlled changes to the QW indium concentrations (see Figure 6). One of the merits of this plot is that it expresses the trade-off between efficiency and current density for a particular colour requirement, expressed by the dominant wavelength.
Figure 5. Wall-plug efficiency (WPE) versus current density (J) for two
representative encapsulated 1 mm by 1 mm InGaN red LEDs with different
wavelength targets. The colour bar indicates the dominant wavelength of
the emission spectrum at each point.
Figure 6. Contour plot of the InGaN red wall-plug efficiency (WPE) as a
function of current density and dominant wavelength at that current
density. The plot is constructed from WPE and spectrum measurements
versus current density for many encapsulated 1 mm by 1 mm LEDs of the
same epitaxy design with varied quantum well indium concentrations.
With red InGaN LEDs, the price to pay for maintaining acceptable colour characteristics at a higher current density is a plummeting efficiency. The workaround is to optimise the epitaxial process for making red LEDs for a very specific current density. There is the potential to exceed a 10 percent WPE at a sufficiently low current density, but LEDs driven in that manner may fall short of radiance requirements. The greatest opportunities to improve red InGaN LEDs through refinement to epitaxy are associated with increasing the IQE, narrowing the spectral width and minimising the blue shift. When driven at low current densities, the operating voltage for the InGaN red LED is already near its theoretical minimum value expected for its emission wavelengths (see Figure 7).
Figure 7. Current density versus voltage (J-V) curves for two
representative InGaN red LEDs with different wavelength targets. The
colour bar indicates the dominant wavelength of the emission spectrum at
each point.
The results described in the previous paragraphs are for ‘macro’ LEDs. The data shows the best performance possible for the epitaxy, using a die design that has a high light-extraction efficiency and is unaffected by surface recombination.
From macro to micro
We have also characterized the performance of a portfolio of microLEDs with various mesa sizes, produced from similar epiwafers. Earlier this year we reported the results of this study at the SPIE Photonics West Conference (see Figure 8 for a summary of our results). This investigation involved the fabrication of arrays with mesas that are all the same size, and connected in parallel to a continuous n-type semiconductor layer. To increase light extraction, we textured the n-type layer after removing the growth substrate. Both InGaN green and blue LEDs were produced for benchmarking purposes. Comparing our range of InGaN LEDs with our AlInGaP LEDs is not straightforward, as a different die fabrication process is needed that may influence efficiency. However, we have made every effort to minimise this impact.
Our results are in line with those of other studies, showing that for InGaN microLED arrays there is a decrease in the sensitivity of efficiency to mesa size with increasing wavelength – and practically zero sensitivity for red. Some have speculated that this behaviour comes from differences in the lateral diffusion length within the QWs depending on carrier localization.
Another important finding of our study is that at a fixed current density the emission profile of the InGaN arrays is basically invariant with mesa size, down to the smallest size studied, which is just 2 µm. This observation is consistent with the conclusions of our finite element analysis, which suggest that the length scale of strain relaxation from the mesa edge is well below 1 µm.
For all colours of our InGaN microLED arrays, we find that the absolute WPE is significantly below that of the corresponding macro LEDs. We attribute this difference primarily to the higher extraction efficiency of the macro LEDs, resulting from their encapsulation and the use of die materials with lower optical losses. However, the efficiency of blue and green microLED arrays is also hampered by surface recombination.
Figure 8. Wall plug efficiency as a function of current density for
microLED arrays of different colours. Solid, dashed, and dashed-dot
lines indicate data in order of decreasing mesa sizes (7 µm, 4 µm, and 2
µm for InGaN; and 9 µm, 6 µm, and 4 µm for AlInGaP). The dominant
wavelengths corresponding to peak efficiency are 605 nm (InGaN red), 630
nm (AlInGaP red), 540 nm (green), and 460 nm (blue).
So long as there is not a hard upper-constraint on the current density of an LED, those made from AlInGaP are capable of a much higher efficiency than their InGaN counterparts, for mesa sizes down to at least 4 µm. What’s more, AlInGaP LEDs have much smaller colour shifts with current density, and their spectra are narrower, with a full-width at half maximum of around 20 nm. However, due to their higher surface recombination velocity compared with their InGaN counterparts, AlInGaP microLEDs have a significant sensitivity to mesa size; to maximize their efficiency, they must be driven at high currents and low PWM duty cycles. To minimise the resulting efficiency trade-offs at the system level, much research and development is now being directed at increasing the internal quantum efficiency of AlInGaP microLEDs at low current densities.
For direct-view applications, such as TVs, which have stringent requirements on the colour characteristics, relatively large pixel dimensions are employed for the displays. Here the advantage of AlInGaP is expected to persist in future, as it is rooted in fundamental physics. LEDs featuring AlInGaP QWs have the intrinsic advantage of a higher radiative recombination coefficient, primarily resulting from the absence of polarization and the QCSE. It’s a different story for head-mounted displays, which require pixel dimensions of no more than a few microns. Based on the extrapolation of our results for both materials systems to smaller pixel dimensions, the indication is that InGaN could be the preferred red emitter material for head-mounted displays, due to the far higher surface recombination losses in AlInGaP.
The case for InGaN may strengthen, as our InGaN red epitaxy is still in its early stages of optimization. We believe there are further opportunities to diminish the droop of InGaN red LEDs and improve their spectral characteristics, drawing on optimization strategies that have brought us success with green LEDs.
Further reading
E. Kioupakis et al. “Interplay of polarization fields and Auger recombination in the efficiency droop of nitride light-emitting diodes”, Appl. Phys. Lett. 101 231107 (2012)
† C-K. Li et al. “3D numerical modeling of the carrier transport and radiative efficiency for InGaN/GaN light emitting diodes with V-shaped pits”, AIP Advances 6 055208 (2016)
† Y. Robin et al. “What is red? On the chromaticity of orange-red InGaN/GaN based LEDs”, J. Appl. Phys. 124 183102 (2018)
† J. Flemish et al. “MicroLED architectures for low-power display applications”, Proc. SPIE 12022, Light-Emitting Devices, Materials, and Applications XXVI, 1202203 (3 March 2022)
† J.M. Smith et al. “Comparison of size-dependent characteristics of blue and green InGaN microLEDs down to 1 µm in diameter”, Appl. Phys. Lett. 116 071102 (2020)