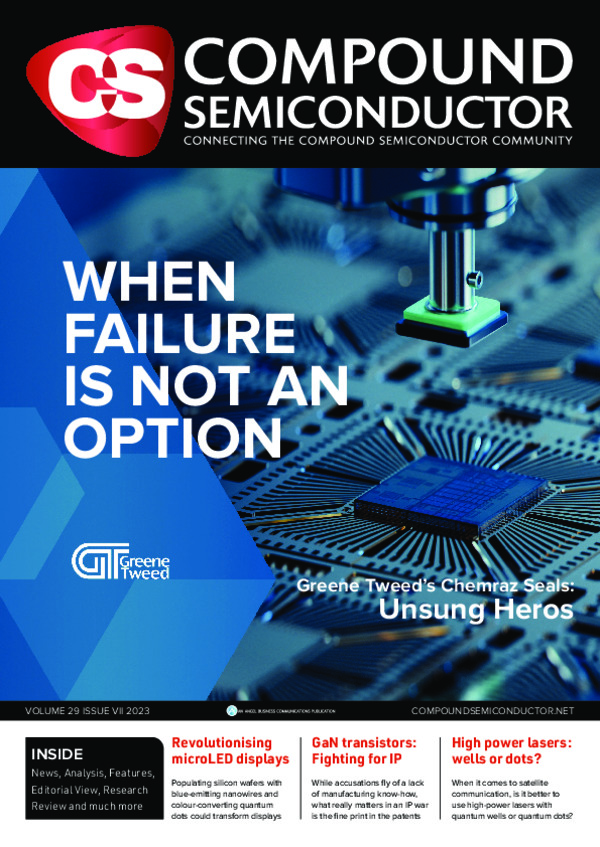
GaN RF transistors 2.0?
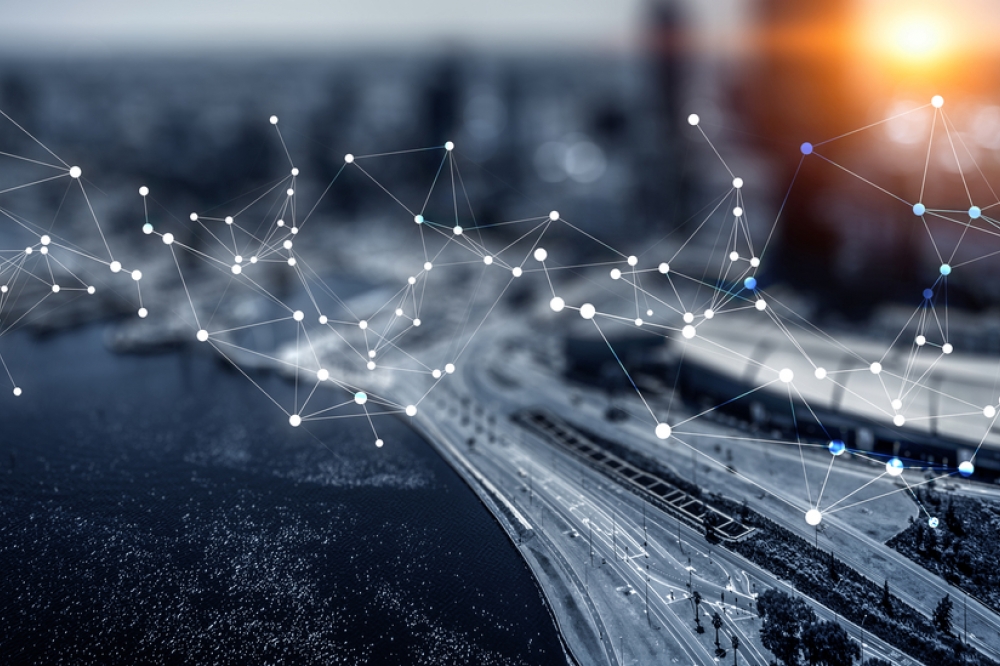
Switching to an N-polar architecture gives GaN transistors greater powers and efficiencies at high frequencies.
BY STACIA KELLER AND UMESH MISHRA FROM THE UNIVERSITY OF CALIFORNIA, SANTA BARBARA
We are living in an era of evolving, diversifying wireless technology. As well as advances in existing technology, such as the introduction of additional standards for mobile communication, new opportunities are emerging, including imaging and autonomous driving. For all these applications, the W-band has great potential. Spanning 75 GHz to 110 GHz and earmarked for NR (new radio), this band is positioned at a local minimum of atmospheric attenuation, so it is well-suited for the propagation of electromagnetic waves.
Options for delivering amplification in the W-band include a number of compound semiconductor technologies. One of the candidates is GaN, which offers higher efficiencies and power densities than GaAs and InP.
In its conventional form, GaN delivers a superior performance to other compound semiconductor materials. However, even better results are possible by switching the polarity of this device. This move has enabled novel deep-recess N-polar HEMTs to outperform traditional Ga-polar technology by more than a factor of two in the W-band. Noteworthy results include a record power density of 8.8 W/mm and a power-added efficiency of up to 31.7 percent at 94 GHz. Another asset of these transistors is the constant high-output power density over a wide range of frequencies. Values are as high as 8 W/mm from 94 GHz down to 30 GHz and 10 GHz – this is a behaviour that’s never been observed for other types of transistor.
The team behind these results is our group at the University of California, Santa Barbara. We have been developing N-polar GaN technology for more than a decade, and over that time we have overcome a number of challenges that have led to performance improvements.
Figure 1. N-polar GaN (circles) provides a breakthrough output-power
density relative to traditional Ga-polar transistors (triangles and
diamonds).
Conventional HEMTs
Conventional GaN-based transistors are well known for delivering high powers at high frequencies. This capability is enabled by the large GaN breakdown field, the high electron velocity and the high two-dimensional electron-gas density (2DEG) accessible in group-III nitride heterostructures. When AlGaN/GaN heterostructures for transistor fabrication are grown in the typical +c-direction, no doping is needed to form the 2DEG, in stark contrast to arsenide and phosphide semiconductors. This is a consequence of the higher ionicity of the Ga-N bond, compared with the Ga-As and Ga-P bond, that causes group-III nitrides to preferentially crystallise in the wurtzite structure, which is non-centrosymmetric in the c-direction. Strong polarisation-based internal electric fields in heterostructures result, giving rise to the formation of a 2DEG at the AlGaN/GaN interface. The Ga-polar (0001) or +c-direction of the GaN crystal is the standard growth direction for group-III nitrides, which is also employed for the production of current optoelectronic devices.
Using standard Ga-polar technology, many academic and industrial groups have produced and improved RF devices. At lower frequencies, such as 4 GHz (S band), Wolfspeed has demonstrated output power densities as high as 41.4 W/mm. Beyond our team at UCSB, an incomplete list of other US contributors to the development of these high-performance devices include HRL, Qorvo, SEDI, Wolfspeed, Raytheon and NGC. Efforts by all have assisted the commercialisation of transistors operating in the S, X and K bands. Now commercially available for more than a decade, these devices are deployed in base stations, radar and satellite communication.
Figure 2. (a) Hexagonal unit cell and (b) atomic structure of Ga-polar
and N-polar GaN. Arrows represent the direction of the spontaneous
polarization dipole, P, in the GaN crystal. Reprinted from S. Keller et.
al. Semicond. Sci. Technol. 29 113001 (2014), with permission from IOP
Publishing, Ltd.
Future applications will involve higher operating frequencies, such as those in the V, E, W, and D bands. Here, the power performance of the standard transistors declines. For many years, power densities for transistors in the W-band have rarely exceeded 3 W/mm, and associated power-added efficiencies (PAEs) have been below 15 percent. To improve transistor performance at these high frequencies, there’s a need to: scale the transistor gate length, enable high currents and good gate control, and ensure a very low access resistance. Departing from the standard Ga-polar transistor structure makes it easier to succeed on all these fronts.
… enter N-polar GaN
To understand the benefits that come from a switch in polarity, there’s a need to take a closer look at the epitaxial layer structure. For the standard Ga-polar transistor structure, which is grown in the (0001) or +c-direction, the AlGaN barrier is positioned on top of the semi-insulating GaN base layer; and the 2DEG channel, which forms at the GaN/AlGaN interface, is located below the AlGaN barrier. In contrast, for transistors that employ the N-polar (000-1) or –c crystal direction, while the semi-insulating GaN base layer and the AlGaN barrier layer are just as they are for the standard Ga-polar structure, the GaN channel is now positioned on top of the AlGaN barrier.
Figure 3. The layer structure of Ga-polar and N-polar HEMTs. In the
N-polar structure the two-dimensional electron gas (2DEG) forms on top
of the AlGaN barrier layer.
There are several advantages that come from this difference in the order of the layers. One is that the 2DEG channel can be contacted via the lower bandgap GaN top layer, making it easier to form low-resistance ohmic contacts. Another advantage is that the 2DEG channel is now positioned on top of the AlGaN barrier, with the latter naturally serving as a backbarrier for carrier confinement, leading to improved gate control. The third significant benefit is that the channel charge can now be controlled independently from the channel thickness, enlarging the parameter space when scaling the transistor, where, in order to maintain good gate control, gate length and gate-channel distance must be scaled simultaneously. (A more detailed discussion of these advantages is provided in M. H. Wong et al. Semicond. Sci. Technol. 28 074009 (2013). While not under consideration here, the N-polar orientation also possesses advantages for E-mode devices.)
The origin of the difference between these two epitaxial structures is that the internal electric fields are in opposite directions. For N-polar heterostructures, the direction of the internal electric field is helpful for a number of device applications. However, drawing on this benefit is far from easy, due to difficulties in the epitaxy of high-quality N-polar III-nitrides.
Figure 4. (a) Schematic of deep-recess N-polar HEMT. (b) Output power
density of N-polar deep-recess HEMTs at different operating frequencies
and drain voltages. The observed independence of the output power on the
operation frequency was never seen for transistors before.
Challenging epitaxy
One common approach to realising an N-polar GaN film begins by taking a c-plane sapphire substrate, which is a well-used platform for GaN epitaxy, and treating the surface with nitridation. This step, undertaken prior to epitaxy, involves exposure of sapphire to ammonia or atomic nitrogen at high temperatures. Unfortunately, no matter if grown on standard c-plane sapphire substrates or on c-plane GaN substrates, for a long time N-polar GaN epitaxial films have been infamous for their hexagonal surface hillocks, thought to originate from inversion domains. In addition, these films contained high concentrations of unintentional carbon and oxygen impurities.
The initial breakthrough came in 2004 when a French team reported the first evidence of smooth N-polar GaN, using C-face SiC substrates and growth by MBE. Soon after this, our team could replicate this success and produce smooth N-polar GaN films by MBE, allowing us to start exploring N-polar GaN based heterostructures for device applications. In 2005 we published our first papers describing the properties of the MBE-grown AlGaN/GaN heterostructures, along with our initial transistor results; and in the succeeding years we have shared the details of our more advanced transistor designs.
We have also investigated the use of MOCVD for growing GaN on C-face SiC. Again, we initially observed unwanted hexagonal surface hillocks. However, we found that the shape and size of these hillocks strongly depends on the growth conditions. This suggested that surface processes influenced the crystal growth, which can be engineered by manipulating the step density on the growth surface via crystal misorientation. It is well known that the larger the misorientation angle from the ideal plane – that is, the perfectly flat crystal surface plane – the greater the number of steps that form on the crystal surface, and thus the shorter the terrace length between these steps. We found that when we grow N-polar GaN films on c-plane sapphire substrates with different surface misorientations, as the misorientation angle increases, the density of the hexagonal surface hillocks declines substantially. Under a wide range of growth conditions, we have produced hillock-free films using a misorientation angle of 4 degrees.
Figure 5. N-polar GaN can serve the full electromagnetic spectrum of interest through D-band.
Note that increases in misorientation angle also improve the structural properties of the N-polar GaN films to the extent that they are then comparable with their Ga-polar counterparts. Another finding is that these smooth N-polar GaN films contain far fewer unintentional impurities. Following optimisation of the MOCVD growth conditions, our N-polar GaN films have residual carbon and oxygen impurity concentrations below 3 x 1016 cm-3. We have also demonstrated N-polar GaN films with properties that are comparable with Ga-polar GaN on misoriented C-face SiC and (111) silicon.
Our development of transistors grown by MOCVD began by investigating device structures for transistors operating at 4 GHz. For this work we initially adopted the epitaxial architecture previously used for our MBE-grown transistors. However, we placed a thin AlGaN layer on top of the GaN channel to mitigate gate leakage. This led to a layer structure of semi-insulating GaN, followed by AlGaN, 25 nm-thick GaN and 2 nm-thick AlGaN.
The performance of these N-polar transistors fully matched their Ga-polar counterparts. We recorded output power densities of 12 W/mm and 20.7 W/mm on sapphire and C-face SiC substrates, respectively. Independent of polarity, the higher thermal conductivity of SiC enabled device operation at higher voltages, and thus higher output power densities. Our results demonstrated the high quality of N-polar GaN/AlGaN/GaN heterostructures grown by MOCVD, and assisted our development of scaled transistors operating at higher frequencies.
To realise transistor operation at 94 GHz, we reduced the GaN channel thickness to 12 nm and introduced a GaN cap layer on top of the epitaxial stack to enable the fabrication of a deep-recess transistor. The GaN top layer delivered two benefits: it moved the surface away from the 2DEG channel, mitigating DC-to-RF dispersion (a phenomenon where the RF performance of the transistor lags behind the one expected from DC testing); and lowered the access region resistance by reducing surface depletion.
Figure 6. (a) Output power density, Po, versus drain voltage, VD, and
(b) power added efficiency, PAE, versus associated output power density,
of N-polar deep recess transistors at 90 – 96 GHz unless labelled
otherwise. The N-polar HEMTs show a higher Po at a given VD indicating
high RF current and excellent dispersion control. The latter together
with the high gain of the N-polar transistors enable their higher PAE at
a given Po.
We reported the results of our first deep-recess N-polar HEMTs on sapphire in 2016, and in the following year the performance of variants on SiC. The latter exhibited a record output power density of 6.7 W/mm and a power-added efficiency of 14.4 percent at 94 GHz. After further optimisation of the device design, we were able to increase the output power density to 8 W/mm at 20 V, and the peak power-added efficiency to 28.8 percent at 16 V. As already mentioned in the introduction, these transistors produce this high output power density over a wide range of frequencies, from 94 GHz down to 30 GHz and 10 GHz, with peak power-added efficiencies of 56 percent and 58 percent at the latter frequencies, respectively.
More recently, we unveiled a power density of 8.84 W/mm (663 mW) with an associated power-added efficiency of 27 percent in 2020; and early this year we announced a single-cell W-band power record of 712 mW, alongside a power density of 7.1 W/mm and a power-added efficiency of 31.7 percent at 94 GHz. Our latest results, announced at the 81st Device Research Conference, are for a transistor with a 1 W output power.
Our body of work demonstrates that compared with standard Ga-polar technology, N-polar deep recess transistors can deliver a higher output power density at a given drain bias, and a higher power-added efficiency at a given output power density. This superiority stems from a high RF current, excellent dispersion control and high gain. The combined outstanding output powers and efficiencies realised with these N-polar deep-recess transistors makes them the leading technology for millimetre-wave applications today – after a nearly 20-year-long journey developing N-polar technology at UCSB.
• The development of the N-polar HEMT technology at UCSB would have been impossible without the hard work of all the graduate students and post-doctoral researchers (too many to acknowledge but all deeply appreciated)
who contributed to the project. This work would also have been impossible without the sustained support of the ONR under contracts supervised by Dr. Paul Maki, sharing the vision that N-polar GaN based transistors could overtake standard Ga-polar technology in performance. Additional support was provided by DARPA under the DREAM program (Drs. Y. K. Chen, Dan Green, and Tom Kazior).
Further reading
E. Monroy et al. Appl. Phys. Lett. 84 3684 (2004)
S. Rajan et al. Jpn. J. Appl. Phys. 44 L1478 (2005)
S. Keller et al. J. Appl. Phys. 102 083546 (2007)
S. Keller et al. Semicond. Sci. Technol. 29 113001 (2014)
C. Lund et al. Semicond. Sci. Technol. 34 075017 (2019)
S. Kolluri et al. IEEE Electron. Dev. Lett. 33 44 (2012)
S. Wienecke et al. IEEE Electron. Dev. Lett. 38 359 (2017)
B. Romanczyk et al. IEEE Trans. Electron. Dev. 65 45 (2017)
E. Akso et al. IEEE Microw. Wirel. Technol. Lett. 33 683 (2023)