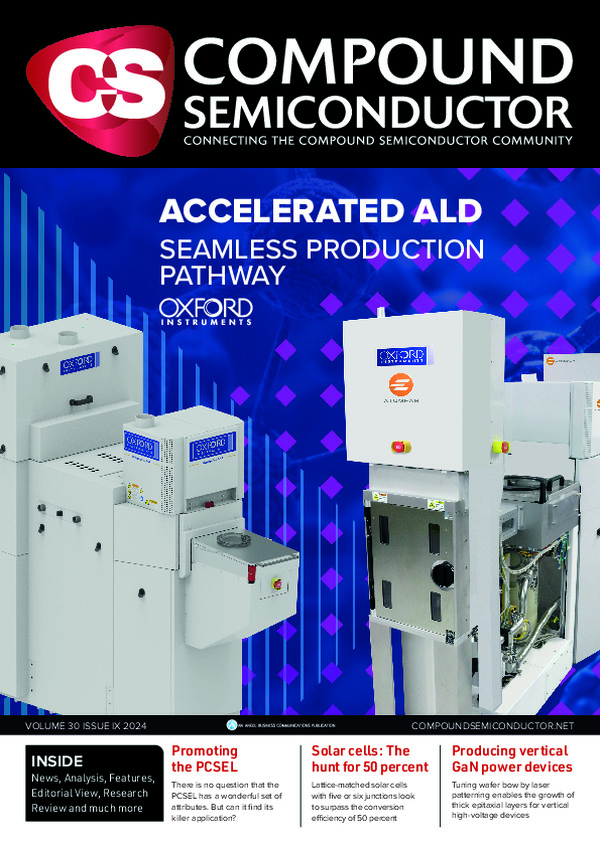
Multi-junction solar cells: The hunt for 50 percent
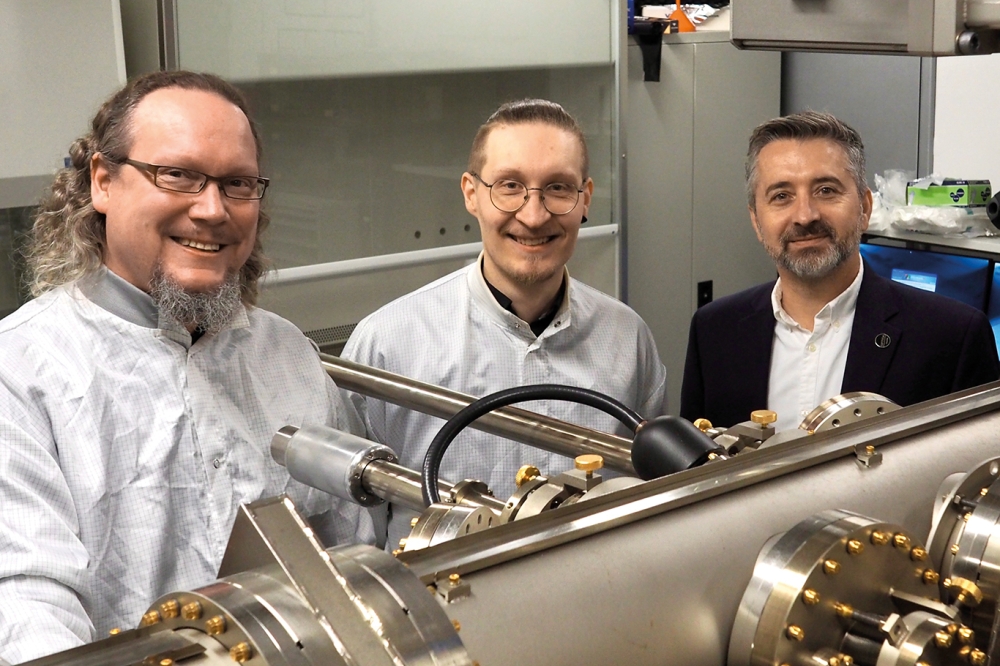
Lattice-matched solar cells with five or six junctions look to surpass the landmark conversion efficiency of 50 percent.
BY MIRCEA GUINA, RIKU ISOAHO, ARTO AHO AND Antti Tukiainen FROM TAMPERE UNIVERSITY
When it comes to photovoltaic conversion efficiencies, III−V multi-junction solar cells are the gold standard. For the past three decades this class of compound semiconductor devices has been breaking record efficiencies and pushing the limits of photovoltaic technologies.
There are opportunities for these multi-junction cells on earth and up in space. Their combination of high efficiencies, high power-to-weight ratios and excellent durability has ensured that these devices are the key technology for power generation in space. Meanwhile, in terrestrial applications, these cells can be deployed in concentrated photovoltaic systems, where focusing sunlight onto the solar cells by lenses or mirrors leads to even higher efficiencies.
Operation under very high levels of concentration, typically many hundreds of suns, is behind record results for efficiency. The current world record stands at 47.6 percent, realised by researchers at Fraunhofer ISE in 2022 with a wafer-bonded four-junction photovoltaic cell operating at a concentration of 665 suns. That’s tantalisingly close to the 50 percent efficiency milestone, which yet remains elusive, despite extensive efforts.
During the last decade or so, realising record efficiencies with multi-junction devices has involved at least four junctions. Now the focus is shifting to five or more junctions, a move that’s considered essential for realising practical candidates that will eclipse the 50 percent target (see Figure 1).
Figure 1. Conversion efficiencies for practical devices.
Unlocking higher efficiencies
Multi-junction solar cells excel in efficiency by dividing the incident solar spectrum between multiple subcells, each absorbing photons at different wavelength ranges. It is the distribution of the incident sunlight spectrum that ensures that radiation is utilised more efficiently, and in turn boosts the overall efficiency of the device.
In principle, the greater the number of subcells, the higher the maximum efficiency. For a purely hypothetical multi-junction solar cell that’s equipped with an infinite number of junctions, the maximum efficiency is 68.7 percent. That figure is calculated for illumination under one sun, with the theoretical maximum of this hypothetical device rising to 86.8 percent under concentration. For a two-junction solar cell the theoretical efficiency under concentration is as high as 50 percent, but it’s likely five or more junctions are needed to reach this value with a practical device.
There are a variety of approaches and materials that can be utilised for realising multi-junction solar cells (see Figure 2). The most widely adopted are stacked architectures based on III−V compounds. III−Vs are a prime choice because of their wide range of tunability, in terms of bandgap energies and lattice constants, assets that enable the monolithic stacking of multiple subcells from different III−V materials.
Figure 2. Different approaches for the fabrication of multi-junction solar cells.
An elegant approach for monolithic integration is the lattice-matched architecture. Initially developed to outperform single-junction GaAs solar cells, this type of III-V tandem solar cell is formed by stacking a lattice-matched subcell, typically made from AlGaAs or GaInP, on top of a GaAs subcell. It’s possible to enjoy higher efficiency by increasing the number of active junctions, a move that’s led to the development of lattice-matched triple-junction cells that are grown on germanium substrates. Note that germanium has a dual role – as well as providing a foundation for the growth of III−V cells, it is utilised as an active bottom subcell. Adopting this approach, Spectrolab raised the bar for efficiency to 41.6 percent under concentration in 2009. This triple-junction design is currently the staple of the space industry, providing over 30 percent conversion efficiencies in space applications, where concentration of sunlight is impractical.
Unfortunately, the triple-junction architecture with lattice-matched designs is not an ideal platform for increasing efficiency through the addition of extra junctions. It’s downside stems from the rather limited availability of traditional III−Vs with suitable bandgaps that can be lattice-matched to GaAs or germanium substrates.
Addressing this weakness requires the development of novel lattice-matched subcell materials. In this regard, one highly promising material system is the dilute nitrides, which can be lattice-matched to GaAs and germanium while offering a bandgap as low as 0.7 eV. Another alternative is to fabricate lattice-matched multi-junction devices on an InP platform; however, the scarcity of suitable high-bandgap materials and the relatively high cost of InP have hindered progress with this approach.
To overcome the limitations associated with lattice-matched materials, some pioneers of III−V multi-junction solar cells have turned to metamorphic designs. This approach relaxes the strict lattice-matching requirements between subcells, opening the door to a far broader selection of materials and optimised bandgap energies, key to improving performance.
However, there are prices to pay for switching to lattice-mismatched materials. There is the threat of compromised crystalline quality and an increase in device thickness. That’s not to say that metamorphic multi-junction architectures cannot triumph – they have achieved notable success, with record efficiencies. Notably, in 2019, the National Renewable Energy Laboratory broke the efficiency record for all photovoltaics, with an inverted metamorphic six-junction solar cell delivering an efficiency under concentration of 47.1 percent.
Figure 3. Improvement of external quantum efficiencies for narrow gap
GaInNAsSb subcells with optimisation of growth temperature and arsenic
overpressure. Insets: Current-voltage curves measured for the GaInNAsSb
solar cells under 900 nm high-pass filtered AM1.5D (1000 W m-2) illumination.
Since then, the record has changed hands and now resides with Fraunhofer ISE. In May 2022 they reported a four-junction solar cell formed with wafer-bonding technology that delivers an efficiency of 47.6 percent. To claim the record, these researchers grew subcells on multiple substrates, before fusing them together to create a two-terminal multi-junction solar cell.
It is also possible to mechanically stack subcells without electrical interconnections in a four-terminal configuration. However, while both this approach and wafer-bonding enable high efficiencies, they are more complex and costly than monolithic integration.
With the 50 percent efficiency target now tantalisingly close, the future of advanced multi-junction solar cells with five or more junctions is highly promising. But what technology will get us over the line? While the inverted metamorphic and wafer-bonded approaches have undoubtedly set high standards for ultra-high conversion efficiencies, it is the lattice-matched approach, bolstered by recent material advancements, that is emerging as a very compelling candidate in the race to reaching the milestone of 50 percent efficiency.
Advantages of lattice matching
The lattice-matched multi-junction architecture offers several advantages over competing technologies, most notably in practicality and cost-effectiveness. Lattice-matching minimises semiconductor material, thanks in part to the absence of thick metamorphic buffer layers. What’s more, there’s no need for a complex substrate removal process, an essential step in the production of inverted metamorphic cells. It’s also worth noting that lattice-matched designs can use identical or similar tunnel junctions between all subcells, further streamlining the design. And last but by no means least, when compared with wafer-bonded and mechanically stacked multi-junction cells, the monolithic lattice-matched approach is simpler and more cost-efficient, as it avoids handling multiple substrates.
In addition to all these strengths, the lattice-matched architecture enhances functionality, particularly for space solar cells. Although lattice-matched designs have been primarily developed on GaAs substrates, it is rather easy to transfer them to germanium substrates, allowing this technology to be applied to germanium-based multi-junction systems standardly used for space applications. In addition, these cells are well-suited for thin-film processing, thanks to reduced internal strain. This functionality enables the fabrication of flexible, lightweight photovoltaic systems with superior power-to-weight ratios, traits highly appreciated by the space industry.
The draw of dilute nitrides
For lattice-matched multi-junction cells on GaAs or germanium substrates, there’s a very limited choice of suitable binary or ternary III−Vs with direct bandgaps. Options are limited to just GaInP, AlGaAs, and Ga(In)As. In essence, this constrains lattice-matched architectures only to the established GaInP/GaInAs/germanium triple-junction designs.
Fortunately, the palette has expanded through developments in novel III−Vs, particularly dilute nitrides. This is a game-changer, giving rise to the opportunity to introduce more diverse multi-junction designs with more junctions.
For those not familiar with dilute nitrides, they are III−V compounds with small amounts of nitrogen incorporated into their lattice, a relatively small modification that significantly alters their material properties. What makes dilute nitrides, such as GaInNAs(Sb) alloys, attractive for solar cells applications is that as they can be grown lattice-matched on GaAs or germanium substrates with bandgaps ranging from 1.4 eV down to 0.7 eV. This wide range of bandgaps, realised while maintaining lattice matching, enables the design of lattice-matched multi-junction cells with better subcell combinations.
The introduction of dilute nitrides to the solar community began with their consideration as a replacement for the germanium junction in triple-junction solar cells. In this role, they can reach the ideal bandgap of 1 eV for the bottom cell.
The downside of dilute nitrides is that they are notoriously challenging to synthesize with sufficient quality for solar cell applications. However, much progress has come from growing this material by MBE, rather than MOCVD. Using this form of epitaxy, epitaxy, the first 1 eV GaInNAs(Sb) subcells have been successfully integrated into multi-junction devices. Highlights of such progress include the former record efficiency of 44 percent with a triple-junction cell incorporating a GaInNAs bottom junction, realised by Solar Junction in 2012.
Our team at the Optoelectronics Research Centre (ORC) at Tampere University has also enjoyed success with dilute nitrides. Back in 2015, we fabricated a GaInP/GaAs/GaInNAsSb triple-junction cell by MBE that produced an efficiency of 37 percent under concentration. This has laid the foundation to our exploration of a pathway to the 50 percent milestone.
To expand the possibilities of lattice-matched designs, we started by developing four-junction cells incorporating two GaInNAsSb subcells. For this design we divide the 1 eV dilute nitride subcell from the triple-junction design into a 1.2 eV GaInNAsSb subcell and a bottom GaInNAsSb junction with a reduced bandgap of around 0.9 eV, realised by increasing the nitrogen content from 3 percent to about 4 percent. This led us to report a lattice-matched AlGaAs/GaAs/GaInNAsSb/GaInNAsSb four-junction cell with a 37 percent efficiency under moderate concentration in 2018. Three years later we announced an improved version with a GaInP top junction and enhanced current-matching that produced 39 percent efficiency under concentration. These successes highlight the potential of lattice-matched multi-junction solar cells with dilute nitride materials for reaching ultra-high efficiencies.
While dilute nitrides with bandgaps of around 1 eV have been successfully utilised in multi-junction solar cells, they fail to cover a significant portion of the infrared spectrum, holding back expansion beyond the four-junction design. To overcome this limitation, we have been focusing on developing lattice-matched narrow bandgap GaInNAsSb alloys for next-generation multi-junction cells with five or more junctions. Our goal has been the development of GaInNAsSb alloys with 0.7−0.8 eV bandgaps that bridge the gap between 1 eV dilute nitrides and germanium.
Figure 4. Left: External quantum efficiencies for subcells in a
prototype GaInP/GaAs/AlGaAs/GaInNAsSb/GaInNAsSb five-junction cell.
Right: Current-voltage curves measured for lattice-matched devices under
500 suns concentration.
It’s far from easy to reach a bandgap of 0.7 eV with dilute nitrides. Nitrogen concentrations have to be pushed to practical limits, creating significant challenges for material quality and epitaxy. But we have made much progress on this front, demonstrating, in 2019, proof-of-concept MBE-grown GaInNAsSb junctions with a bandgap of 0.73 eV and a nitrogen concentration of around 8 percent. These cells maintain crystalline integrity, but high nitrogen levels degrade their optical and electrical properties.
Recently, we have realised more promising results with 0.8 eV GaInNAsSb junctions containing about 6 percent nitrogen (see Figure 3). This slight reduction in nitrogen composition, combined with further optimisation of MBE growth, significantly improves photocurrent from this bottom cell by reducing background doping levels. These optimised 0.8 eV GaInNAsSb junctions are already suitable for integration in multi-junction devices, potentially enabling efficiencies that would break the 50 percent barrier.
Drawing on this success, our next logical step has been the pursuit of lattice-matched multi-junction cell designs incorporating either five or six junctions and leveraging these novel materials. We have already developed prototypes for both cells (see Figures 4 and 5).
Our five-junction variant builds on our the four-junction design, incorporating an additional AlGaAs subcell between the GaInP and GaAs top cells. Early results reveal promising open-circuit voltages exceeding 5 V under concentration, nearly 1 V higher than those of the four-junction cell. However, the bottom dilute nitride junction limits the current, resulting in a conversion efficiency of approximately 30 percent. Realising the targeted current density of around 9 mA cm-2 with further optimisation of this five-junction cell, increasing efficiency to around 45 percent under concentration is not unrealistic.
For our six-junction prototype, we incorporate three dilute nitride subcells, employing the newly developed 0.8 eV GaInNAsSb subcell as the bottom junction. To enhance current-matching between the subcells, we adjust the bandgaps of the two other GaInNAsSb subcells to higher energies than those adopted in the five-junction design, while maintaining the same bandgap configuration for the top three junctions. Although device integration is still in its early stages, experimental results are promising, with open-circuit voltages around 5.5 V under concentration. However, similar to the five-junction prototype, the current-matching target is yet to be realised, limiting the experimental efficiency to approximately 30 percent.
Figure 5. Cross-sectional scanning electron microscope image (left) and
reciprocal space maps (right) for a prototype lattice-matched
six-junction solar cell produce at Tampere University.
Fulfilling their full potential
Although our prototype five- and six-junction cells demonstrate the potential of the lattice-matched approach for next-generation multi-junction devices, there is still significant room for optimisation. Most notably, in order to realise the targeted current values, we need to carefully tune subcell bandgaps and thicknesses to optimise current generation. This is far from trivial, because increasing the number of junctions makes current matching increasingly challenging and precise.
Turning to advanced processing techniques helps to further enhance the performance of multi-junction devices. Within this toolkit is the anti-reflective coating, added to minimise reflectance across the entire spectral range of the solar cell. This must be applied with great care, as the design of the coating impacts current balancing between the subcells. Success necessitates meticulous tailoring for optimal device performance. In practice, this tends to involve the application of optimised multilayer coatings with increasing complexity. Our prototype five- and six-junction cells already employ six-layer dielectric anti-reflective coatings. We also have the option of using nanostructured coatings, which provide low reflectance over a wide spectral bandwidth.
To expand the application of multi-junction cells to high-value specialised areas where flexibility is a benefit or even a requirement, these devices can be processed as thin-film structures using epitaxial lift-off (ELO). It is a technique already deployed for the fabrication of thin GaAs-based solar cells with fewer junctions, motivated by the need to increase the power-to-weight ratio in space power systems and unmanned aerial vehicles. Another benefit of ELO is that it enables substrate re-use, thereby providing major benefits in terms of the sustainability of critical materials.
Start-up tf2 devices, a spin-off of Radboud University Nijmegen, has demonstrated the application of ELO to a four-junction photovoltaic cell. However, converting this research result to wide-scale production is yet to take place, much due to the complexity of the process. One issue that has to be tackled is that typical multi-junction solar cells incorporate a high degree of strain, making the lift-off process structure dependent and potentially resulting in structural damage once the thin-film active device is removed from the substrate.
Benefiting from the lattice-matching of our structures, we are advocating a new method that ensures higher controllability of film curvature, realised through controlling the strain within the structure. A key element in our process is the use of an InGaAs/GaAs stressor layer, grown beneath the solar cell, that helps control the extent of the strain required to roll-out the thin film (see Figure 6). An external polymer stressor layer can be added to enhance control over lift-off.
We have already demonstrated our ELO technique for a single-junction GaAs cell, and we are now working on extending the process for multi-junction structures. They have an increased thickness, and precise strain management is required across different subcells to ensure a controlled self-rollup.
Another of our goals is to develop methods for fabricating electrical contacts for thin-film solar cells utilising inkjet-printed polymers. An alternative to wire bonding, our printing-based approach promises to improve the handling of thin-film structures and boost cell performance, while potentially trimming manufacturing costs.
The thin-film configuration also offers another advantage: it enables the fabrication of back reflectors, enhancing the current generation and radiation resistance of space solar cells. Our lattice-matched six-junction design would greatly benefit from a back reflector, as it’s addition could enhance current generation in the novel narrow-gap GaInNAsSb bottom junction. We have extensively developed back reflectors for III−V solar cells, with both planar and textured structures applied to dilute nitride and quantum-dot solar cells.
Figure 6. Illustration of the epitaxial lift-off (ELO) process for a
structure with an internal stressor layer (left), and a picture of an
experimental self-peeling GaAs solar cell with inkjet printed polymer
used for making the contacts (right, credit: Prabudeva Ramu).
To produce lattice-matched multi-junction designs with a higher number of junctions that incorporate better bandgap configurations, there is a need for III−V subcells with bandgap energies exceeding 2 eV. Two suitable candidates are AlGaInP and AlGaInAsP. However, it is a challenge to fabricate high-performance subcells with these compounds, due to issues related to impurities, doping, and carrier mobilities. The well-established path to realising higher bandgap energies is to introduce higher aluminium compositions, but that exacerbates these issues.
Figure 7. Left: Materials and material systems suitable for
lattice-matched multi-junction solar cells on GaAs and germanium. Right:
Current densities of experimental AlGaInP solar cells grown at Tampere
compared to the current density targets of five and six junction cells.
Taking on these challenges, a team from NREL has successfully utilised a 2.1 eV AlGaInP layer in its metamorphic six-junction cell. However, the addition of this higher bandgap layer increases series resistance. Our preliminary AlGaInP junctions show similar limitations, but we estimate that AlGaInP junctions with aluminium concentrations of between 10 percent and 26 percent could potentially realise current-matching in lattice-matched five- or six-junction designs, provided that further research addresses the challenges with high series resistances, improving their performance under high concentrations (see Figure 7).
Such success would be another step on the way to breaking the 50 percent barrier. It’s a goal that’s tough, but far from impossible.