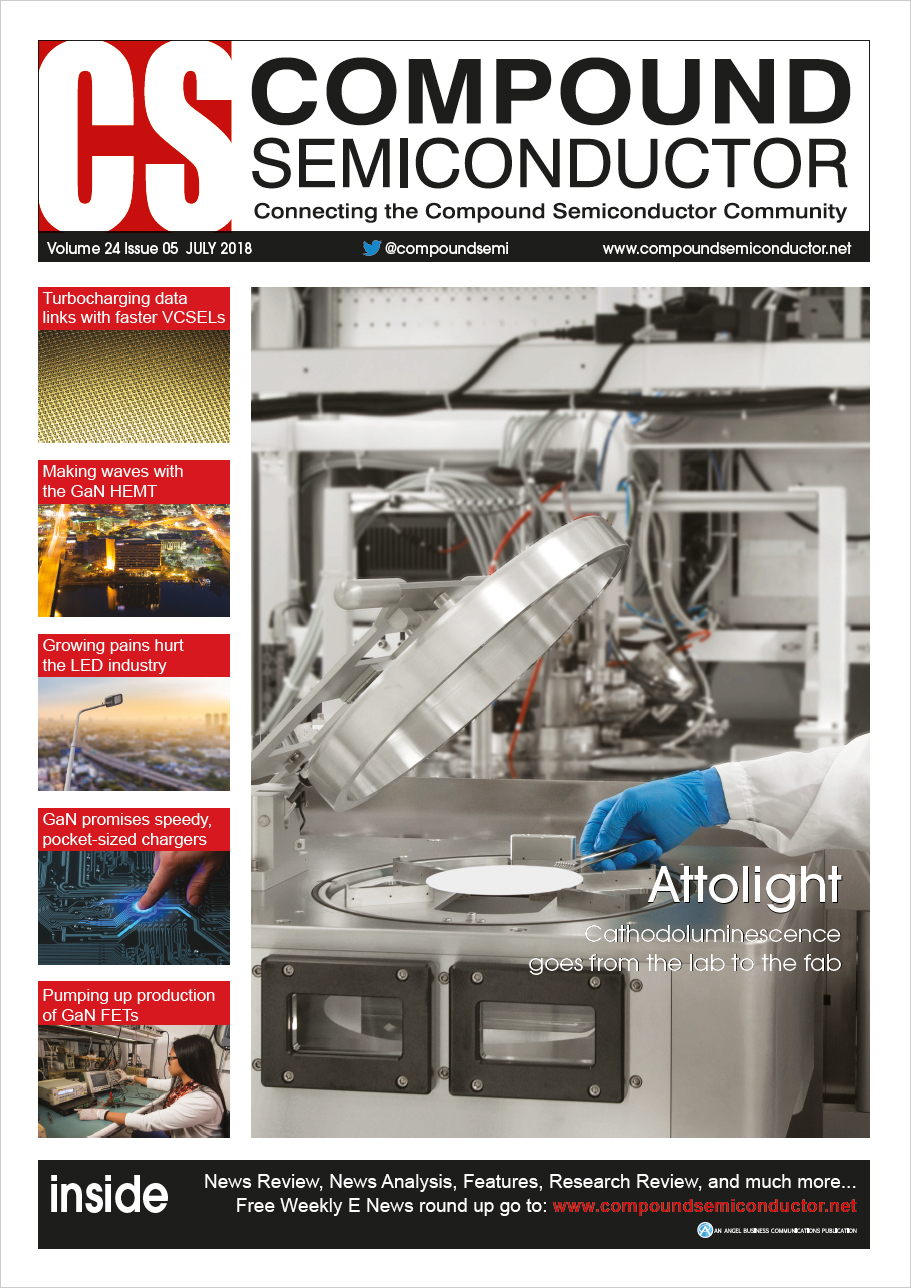
Cathodoluminescence for high-volume manufacturing
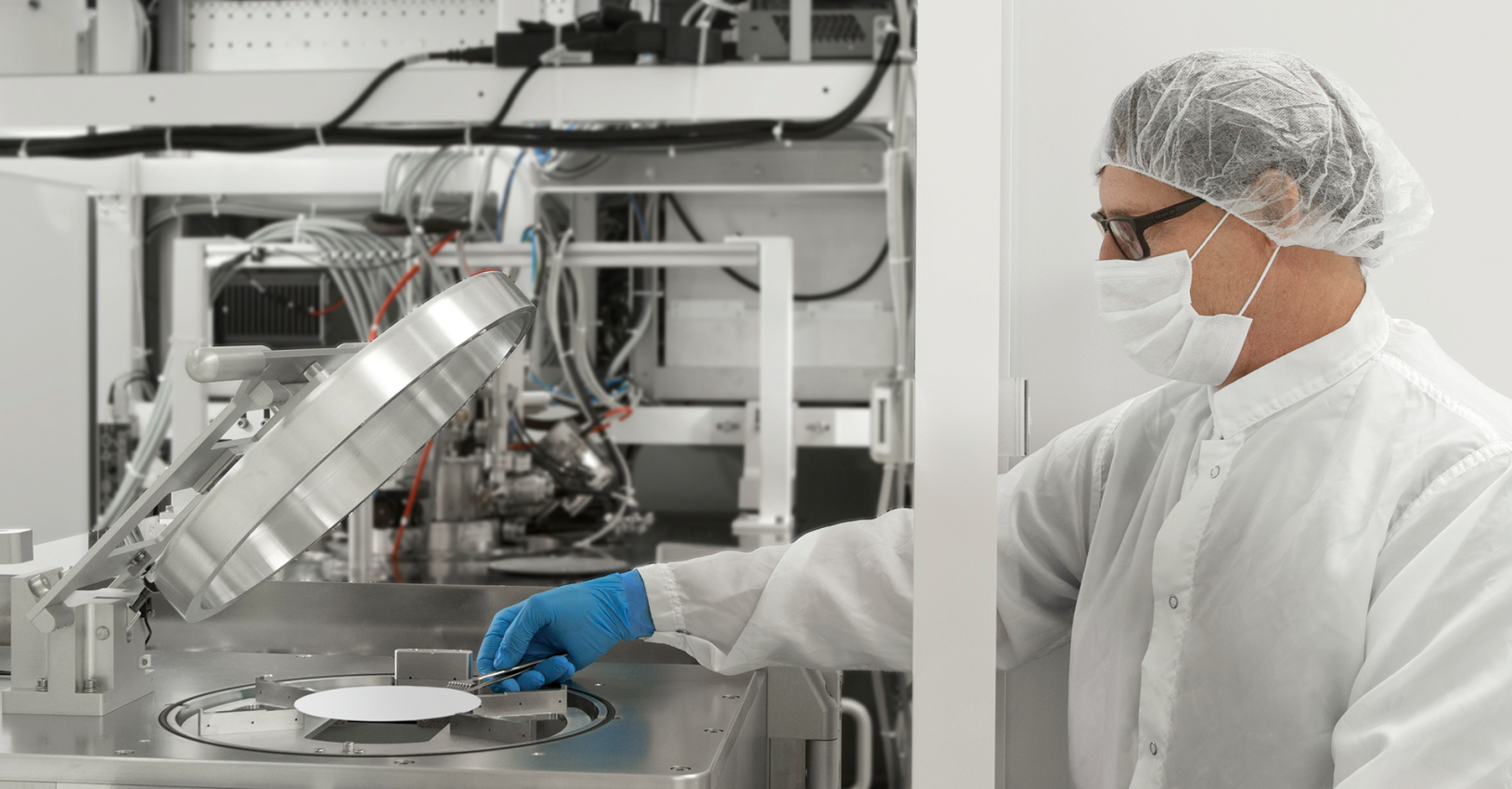
Our industry prides itself on the production of countless high-quality, reliable devices, which can be degraded at the nanoscale by threading dislocations, stacking faults, inclusions and point defects. Often these imperfections arise during the epitaxial process, due to differences in the lattice or thermal expansion coefficients between the substrate and subsequent layers. However, they can go unnoticed until the end of the line, or even appear in shipped product, where they pose a serious threat to the device's capability and reliability.
If unnoticed, a killer defect can turn out to be incredibly costly. It is not just the expense associated with undertaking unnecessary production steps on a chip that is destined for failure. The cause of the defect may also need tracing "“ and when it is exposed, it can reveal a drift in the manufacturing process, and a need to scrap much material. There is also the worst case scenario: chips fail in the field, resulting in angry customers that take their business elsewhere; and the need for expensive failure analysis.
What's needed is a reproducible, non-destructive defect inspection method that is fast enough for use in production. And at Attolight of Lausanne, Switzerland, we have a cathodoluminescence tool that does just that, the Säntis 300.
Cathodoluminescence is a characterisation technique that involves directing a beam of electrons at a sample, and recording the light that is emitted from it. Much can be resolved, because the electron beam can be focused down to a few nanometres. This allows a sample to be mapped with nanometre resolution, beating light's diffraction limit by several orders of magnitude. What's more, cathodoluminescence can be spectrally resolved, offering tremendous insight into material properties (see Figure 1).
Cathodoluminescence is even capable of detecting threading dislocations, a defect responsible for short circuits in power transistors. This may raise a few eyebrows, given that a threading dislocation has a diameter of an atom, and normally it cannot be resolved by an electron probe that is one or two orders of magnitude larger. But cathodoluminescence offers unparalleled sensitivity to defects, due to the light emission process.
In the cathodoluminescence process, electrons that hit the surface subsequently impinge on the semiconductor, slow down and undergo multiple scattering events. Every time there is scattering, electrons within the semiconductor are excited from the valence band to the conduction band to form electron-hole pairs. These pairs eventually recombine, emitting photons in the visible range.
As the cathodoluminescence emission spectrum is directly related to the difference in energy between the valence and conduction bands, it is extremely sensitive to the electronic band structure "“ and ultimately extremely sensitive to any atomic change or atomic defect. A mere vacancy or dopant inclusion can disrupt the material's band structure over length scales up to tens of nanometres. Consequently, even though a defect may be far smaller than the electron probe, it affects cathodoluminescence over tens of nanometres, and its presence can easily be detected with a state-of-the-art cathodoluminescence scanning electron microscope.
The emission energy of the cathodoluminescence depends on the nature of stacking faults, local strain variations and point defects, such as dopant inclusions or vacancies. Threading dislocations act as non-radiative recombination centres, quenching cathodoluminescence. Defect sensitivity can be as good as 1016 atoms cm-3, making this, to our knowledge, the most sensitive non-destructive method for uncovering defects.
It is easy to see why cathodoluminescence's high sensitivity to defects makes it a great technique for performing failure analysis and R&D characterization. However, in a high-volume manufacturing environment, how can it be practical to sample a full wafer every ten nanometres? The good news is that it doesn't have to be this way, thanks to cathodoluminescence's greatest weakness becoming its advantage: carrier diffusion.
Figure 1. Acquisition of a cathodoluminescence map. The electron beam scans the surface of a wafer. At each point, light is emitted, spectrally resolved so that a hyperspectral map (a map made of multiple colours) is measured.
Carrier diffusion: a curse to a blessing
When electron-hole pairs recombine at the place of generation, cathodoluminescence stems from the precise location of the focused probe. But in a defect-free semiconductor that's not always the case, as electron-hole pairs can diffuse over hundreds of nanometres before recombining.
At first glance, that suggests that electron-hole pair diffusion reduces the image resolution. But that's not the case.
Consider a killer defect, such as a threading dislocation. The defect disrupts the local electric field, and traps electron-hole pairs that are nearby. As these pairs cannot diffuse or recombine radiatively, local cathodoluminescence is quenched. For electron-hole pairs generated further from the defect, the chances of diffusing towards it are far less, making radiative recombination more likely. Due to these factors, defects appear in cathodoluminescence images as very small dark spots, limited by the probe size and interaction volume, that fade away over a few hundreds of nanometres.
Sometimes defects may radiate. However, they will always modify the carrier wave function in their vicinity, and provide a distinctive cathodoluminescence spectrum. This may be used to identify a defect or a population of defects, even if they have an atomic size.
Note that a map can even be acquired by spacing out measurement points by hundreds of nanometres. So long as the spacing between measurement points is smaller than the diffusion length, the mapping technique will uncover defects. It is this feature that makes cathodoluminescence the ideal low-sampling technique for spotting loosely distributed defects without having to destroy the sample.
Figure 2. (left) Spectra from point 1 (on a dislocation) and point 2 (stacking fault). (centre) Secondary electron microscope map acquired simultanously to (right) hyperspectral map, having a colour for each defect band. The blue band shows threading dislocations, the green and red band show stacking faults on a GaN template.
A poor reputation
Cathodoluminescence is not a new technique. The phenomenon was first reported as far back as 1879, and cathodoluminescence microscopy has been known since the 1960s. However, use of this technique has been limited to the laboratory, where it has a poor reputation, due to the lack of dedicated instruments.
While collecting cathodoluminescence emitted by the sample is simple in principle, it is not in practice. Difficulties occur, because in the electron microscope that provides the well-defined electron beam, there is competition for the same space by the objective lens of the light and that of the electron microscope.
Up until now, the solution has been to insert a conical mirror, in either a parabolic or elliptical form, into an existing electron microscope.
Figure 3. Wafer map showing a wafer with the user defined measurement locations.
One of the many downsides of this approach is that the conical mirror exhibits significant off-axis aberrations, impairing imaging when the wafer is probed anywhere except at the exact focal point of the mirror. Aberrations are so significant that light emitted from the edges of the cathodoluminescence map hits the aperture stop, so it is clipped before reaching the detector "“ a measurement artefact called vignetting.
Additional drawbacks are that: secondary electron detection is affected by the mirror, making it hard to reach the microscope's ultimate resolution; and electron microscopes are not designed to work at the optimal conditions for cathodoluminescence, which are a low beam energy and a high current. Instead, a high electron beam current is often traded for a very high resolution at low current.
All these issues have hampered cathodoluminescence, which has a reputation for a lack reproducibility and stability, and lengthy alignment times. These drawbacks motivated us to build the first dedicated cathodoluminescence scanning electron microscope, the Allalin, and its fullwafer counterpart, the Säntis 300.
The latter breaks new ground by bringing cathodoluminescence to high-volume semiconductor production. It has been constructed to produce the highest resolution cathodoluminescence maps possible, and deliver an unprecedented signal-tonoise ratio. Measurement physics, not technology, is the tool's ultimate limit.
Fantastic features
One of the key features of the Säntis 300 is its entirely new cathodoluminescence collection objective, which has zero off-axis aberration and zero photon loss "“ allowing for perfectly uniform, reproducible, and quantitative measurements with the highest possible collection efficiency. This approach eliminates alignment time and maximises the signal-to-noise ratio to its physical limit "“ no matter where the electron probe is placed.
Another attribute of our tool is its state-of-the-art field emission gun technology. The advanced gun trims aberrations by a factor of three and produces higher brightness. A higher probe current is used without compromising resolution. This helps us to dramatically improve the signal-to-noise ratio, speeding cathodoluminescence measurements of weakly emitting specimen, and in some cases making it possible to image a sample.
Figure 4. Full-wafer brush image of a 150 mm LED wafer (left) and red-green-blue cathodoluminescence map representation (right)
One of the potential pitfalls of a high brightness gun is that it can introduce unwanted electron-electron interactions. To prevent this, we use a very simple, elegant design that ensures electrons are separated from one another at the source, and don't interact as they travel to the sample.
We also employ an electron microscope objective lens that leaves a large opening, to allow the inclusion of a high-numerical-aperture light microscope that collects as much light as possible. Our tool is designed so that the optimum focal plane of the electron microscope matches the focal plane of the light microscope. With this approach the user can always work with the smallest probe size.
To realise mapping with sub-micrometre resolution, the beam energy in a cathodoluminescence tool must be below 10 keV. We adhere to this requirement, optimising the objective lens of the electron microscope to deliver a massive electron beam current in the 3 keV to 10 keV range. This is accomplished while maintaining a probe size below 10 nm, to ensure that it takes very little time for users to produce high resolution cathodoluminescence maps.
Figure 5. (left) Dark spots corresponds to threading dislocations on a GaN template. (right) Lines represent stacking faults, the dark spot close to the centre is a threading dislocation on SiC
When we set out to design a quantitative cathodoluminescence detector, one of our primary goals was to maintain the high collection efficiency and spectral resolution when steering electrons away from the optical axis. To accomplish this, we designed a high numerical aperture reflective objective that is optimized to fit in an electron magnetic lens and excel on three fronts: in achromatism, ensuring freedom from colour-related distortion; in aplanatism, to eradicate image distortion; and in thermal characteristics, so that the objective is stable with regard to temperature changes.
Another attribute of this reflective objective is that it avoids the tiny aperture stop, which standard systems use to achieve high spectral resolution at the expense of low light throughput and vignetting.
Figure 6. (right ) Graph showing changing silicon-dopant concentration in a staircase GaN structure measured with high precision using cathodoluminescence. In addition, the precise positioning of the electron beam allows to extract optical signatures of small sized areas within a device, non-destructively in top view, or on cross sectioned samples. (left) CL map of the staircase structure.
Thanks to its great design, the Säntis 300 can produce cathodoluminescence intensity maps at a rate of 100 ns per pixel, while realising a dynamic contrast of two-tothree orders of magnitude with materials with adequate emission efficiency, such as III-Vs with a direct band gap. This throughput is sufficient for characterising layers in a high-volume semiconductor fab.
It is worth noting that the Santis 300 system is capable of more than just cathodoluminescence. In its most powerful configuration, it also offers: scanning electron microscopy imaging; UV-visible and nearinfrared hyperspectral imaging, including an individual pixel optical signature; and panchromatic fast imaging (see Figure 2 and 8 for simultaneous scanning electron microscopy imaging and hyperspectral imaging).
Figure 7. Cathodoluminescence spectrum of 1.25 nm thick AlN etch stop layer. The Säntis 300 is UV capable and sensitive enough to measure spectral signatures over very thin layers that emit photons in the UV spectral range.
Modes of operation
Another feature of the Säntis 300 is its three acquisition modes: step and repeat, across wafer pixel, and full wafer brush.
The step and repeat mode is the one that is most likely to be used in a high-volume fab for non-destructive control of buried defects. When the tool operates in this mode, the user specifies a wafer map by predetermining a list of measurement locations (as shown in Figure 3) that will be investigated and specifies acquisition parameters. The tool then sequentially loads wafers, focuses the electron beam onto them and acquires, simultaneously, secondary-electron and cathodoluminescence maps at each measurement location. Wafers are repeatedly characterized, allowing statistical process control at the highest possible spatial resolution for characteristics such as the distribution of defects, their size, and their spectral signature.
If engineers need to carry out in-depth analysis, they can produce hyperspectral cathodoluminescence maps from wavelengths as short as 200 nm in the UV to 1700 nm in the mid-infrared, using over a thousand channels "“ or colours "“ per pixel. Alternatively, they can acquire fast single-channel maps.
Running in the across wafer mode, the Säntis 300 operates with a medium electron-beam spotsize and produces cathodoluminescence intensity maps of full wafers or partial areas of wafers. Operating in this manner takes full advantage of optical signature acquisition. Variations of material across the substrate can be detected. Two hours are needed to map a 150 mm wafer, and partial scanning is proportionally faster.
Engineers will primarily use the across wafer mode for non-destructive process development. This includes efforts at yield improvement, which target uniformity characterization. Studies are related to the likes of buried defect density and impurity variations. Success that results from this approach includes faster development cycles and shorter ramping times.
Operation in the third mode, full-wafer brush, involves defocusing the electron probe and measuring the whole wafer with a low spatial resolution "“ it is 300 μm. Full 150 mm wafers can be measured in about 13 minutes. This approach generates a massive hyperspectral map of the wafer, enabling a measurement of the variation of material composition across the substrate (see Figure 4).
One of the powerful features of the full wafer brush mode is that it allows an engineer to set upper and lower control limits on the input signal "“ this enables automatic identification of step-and-repeat locations, which require high-resolution characterization. Operated in this manner, the statistical process control of the Säntis 300 can be extended from optical characteristics and image analysis to X-Y location acquisition coordinates. This allows process profile wafer mapping to be inferred and used as an additional parameter for non-destructive production control, with a fast feedback loop to the process tool.
A versatile tool
As defects are an issue for the production of any device, our Säntis 300 can make a valuable contribution in fabs that are manufacturing LED, microLEDs, SiC and GaN power and RF chips, photovoltaics, and III-V photonics. For all these devices, substrates and epitaxial layers are the base of the pyramid on which devices are built. It is challenging to detect sub-surface defects with nanometre resolution on substrates with diameters up to 300 mm, but the Säntis 300 is up to the task: it can specifically recognize, isolate and quantify most of the defects of interest, including buried defects.
Figure 8. Intermediate process check. (left) Secondary electron map measured simoultanously to (right) hyperspectral cathodoluminescence map showing wavelength shifts over more than 700 microLED structrures.
Our tool is capable of rapid measurements and automatic counting of threading dislocations in a GaN or SiC blanket layer, for densities up to 5 x 109 cm-2. In the example shown in Figure 5, 882 dislocations are identified in a scan area of 25 μm by 25 μm, corresponding to a density of 3 x 108 cm-2.
Even if a layer does not emit light, it might absorb light coming from sublayers and could be indirectly observed this way. This issue can be addressed by cathodoluminescence mapping where the Säntis 300 provides pinhole detection of a non-luminescent layer on top of a luminescent layer.
The optical signature returned by cathodoluminescence is heavily influenced by the type of impurity, and by the level of doping or the impurities in a material. However, characterisation of doped areas is possible with the Säntis 300, according to cross-correlation studies with other techniques, such as secondaryion mass spectrometry (see Figure 6, variations in the emission peak can directly be correlated with the doping concentration. The silicon-dopant concentration can be inferred with high precision).
Thanks to the precise positioning of the electron beam, optical signatures can be taken from smallsized areas within a device. This can be a top-view, or if destruction of the sample is permitted, a profile of the cross-section.
Plasma etching plays its part in the manufacture of many devices. Unfortunately, it can introduce defects on landing layers or impurity loading on a sidewall. These imperfections can be picked up with the Säntis 300, which can also assess the integrity of an etch stop layer (see Figure 7).
One of the most challenging devices to characterise is the micro-structured LED array. That's because conventional optical methods have insufficient resolution. What's more, a series of process steps produce a structure that is influenced by interactions that cannot be accounted for during the characterization of a particular step.
Addressing these issues is the Säntis 300, which can perform the critical intermediate process checks. In addition, it allows engineers to determine process uniformity over large fields with high spatial resolution "“ this holds the key to yield improvement and faster process development (see Figure 8 for an example of imaging microLEDs with the Säntis 300).
For emitting devices, such as LED and lasers, a full wafer scan can be performed using pre or post singularizing of the dies. During this measurement, undertaken at either room temperature or at the functioning temperature of the device, the electron beam activates the device, while optical collection fully characterizes the wavelength, intensity and other parameters of interest. Adopting this approach allows rapid sorting of the die.
Our examples of the use of the Säntis 300 offer an insight into the versatility and importance of quantitative cathodoluminescence. We believe that the launch of this tool promises to revolutionise material characterisation in compound semiconductor fabs all over the world.