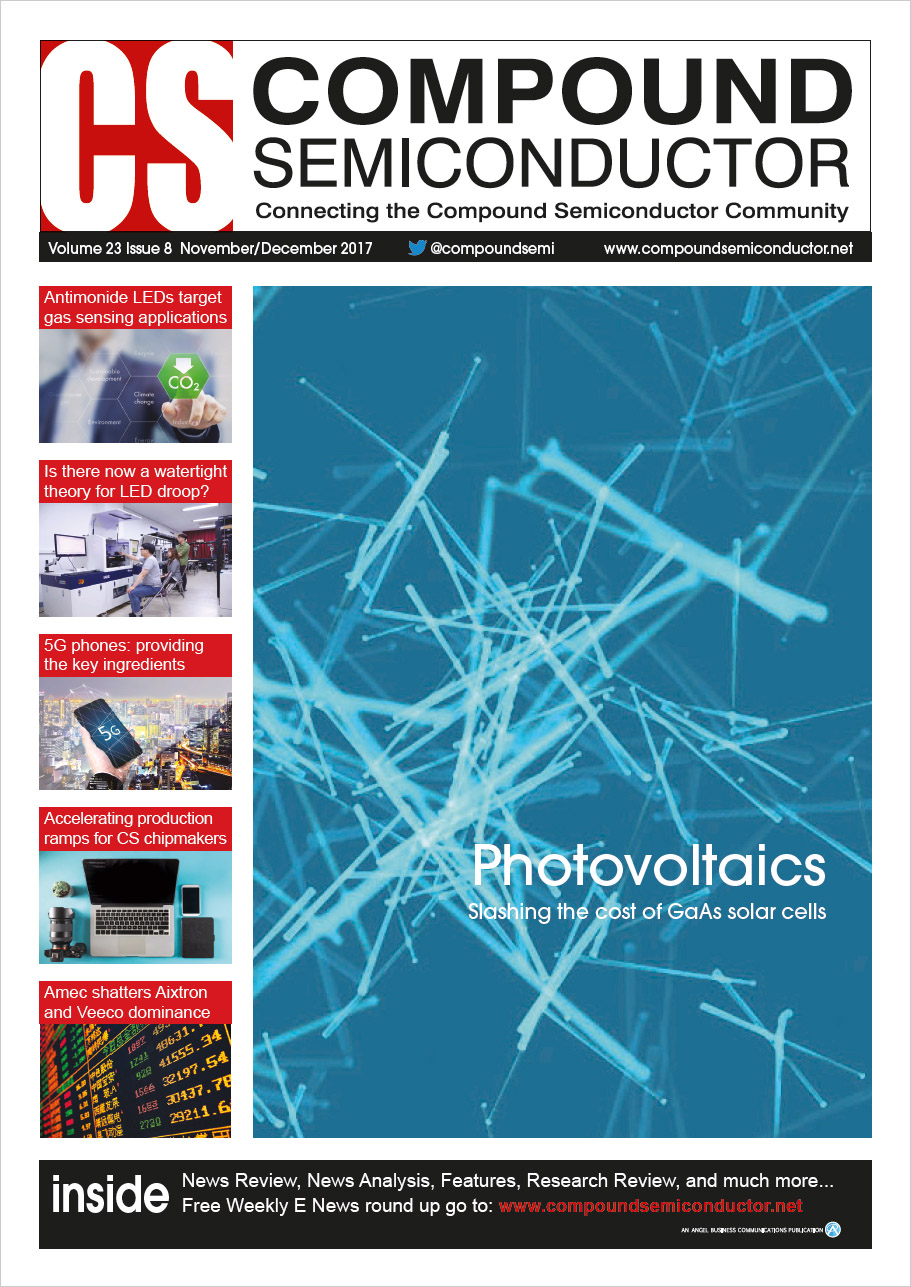
GaAs provides the key ingredient for 5G phones
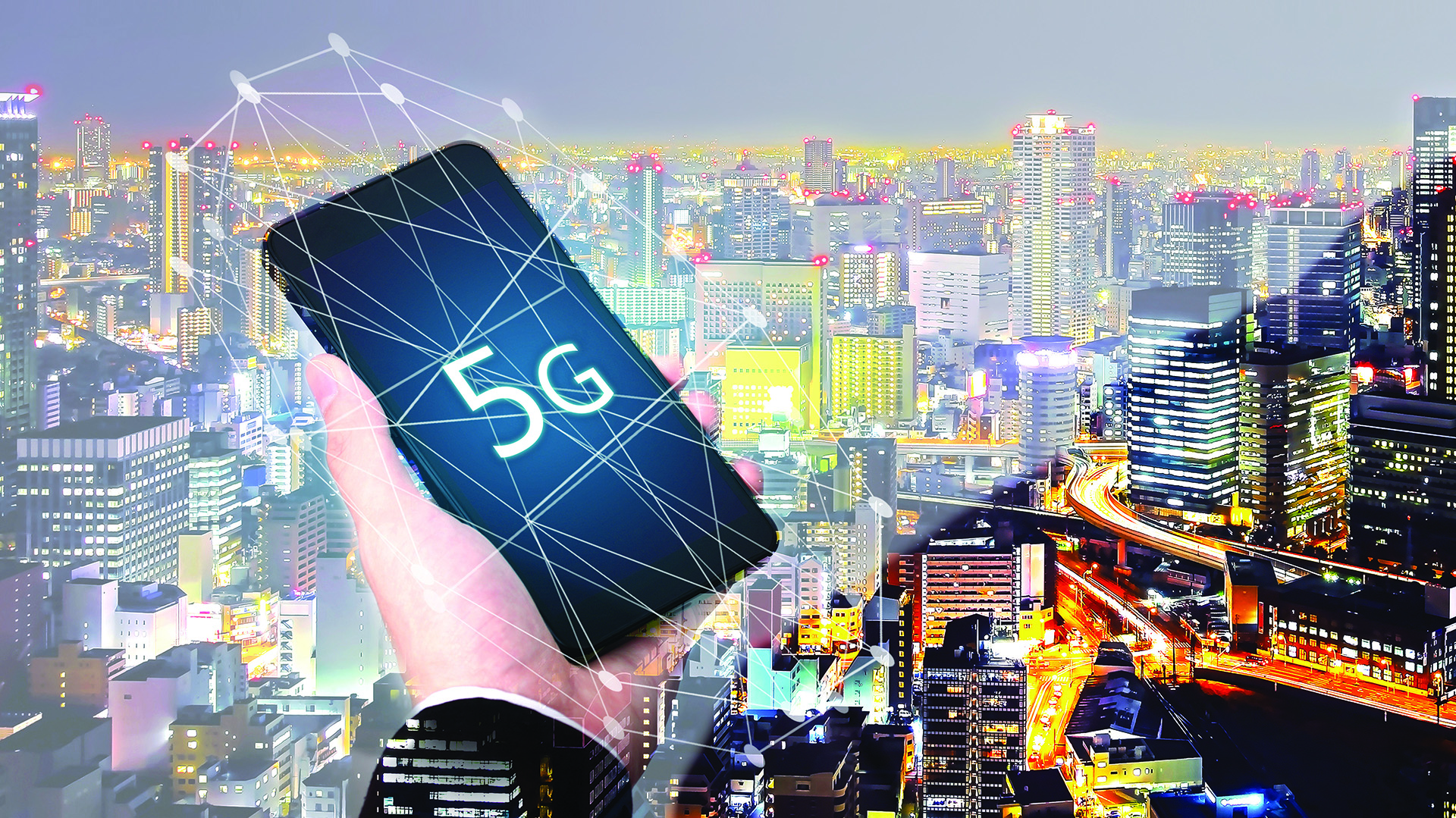
GaAs can meet all the requirements for the power amplifiers used in front-end 5G modules by Ben Thomas from Qorvo
When will the roll out of 5G begin? Until recently, that was anyone's guess. However, the timing has now become clearer, and it could be as early as 2019.
Driving the acceleration in the deployment of this communication technology is a recent advance in global broadband standards: the non-standalone radio. It enables operators to deliver 5G data speeds more quickly and affordably, via an enhancement to existing 4G infrastructure, rather than a replacement. There are implications, though, including complex new challenges for mobile RF front-ends. They will need to support 4G and 5G waveforms, unprecedented bandwidth and new high-frequency bands.
These efforts will be worthwhile, because when the build-out of 5G networks takes place, it will make a significant impact. It will lead to: enhanced mobile broadband for smartphones and other mobile devices, which will enable carriers to provide much higher peak data rates and expand network capacity to support more users at higher densities; ultra-reliable, low latency communications for critical applications requiring very low latency, such as self-driving cars and industrial control systems; and massive machine type communications for a vast number of Internet-of-Things devices that require low-power, relatively low-bandwidth communications.
To realise all of this, 5G networks will use a much wider range of frequencies than today's 4G networks, including millimetre-wave frequencies. And to support the divergent requirements of the differing applications, they will adopt a technique known as network slicing, which allocates to each device a varying degree of latency, data rate and security level, based on their specific needs.
When it comes to enhanced mobile broadband, 5G will complement 4G, rather than replacing it. Operators are already enhancing their existing 4G networks with LTE Advanced and LTE Advanced Pro capabilities. This should lead to peak data rates of up to about 1 Gbit/s via carrier aggregation (CA), a technique that combines multiple data carriers and leads to greater bandwidth. However, to meet the anticipated growth in mobile broadband, networks will need to combine even greater capacity with higher speeds than LTE can provide.
An indication of the extent of these increases in capacity and speed is given by the predictions provided by the Cisco Visual Networking Index. According to this source, data consumption for the average smartphone user is expected to quadruple to nearly 7 GB per month by 2021. Most of that growth will be driven by video traffic, together with emerging applications, such as augmented reality and virtual reality. To support these capabilities, 5G enhanced mobile broadband is planned to deliver peak network data rates of up to 10 Gbit/s.
The pressure to accelerate the 5G standards process, which will be driven by these advanced capabilities, culminated in the definition of non-standalone radio in March 2017. The mobile standards organization 3GPP agreed to divide the development of the 5G new-radio specifications into two phases. The non-standalone specification, designed primarily for mobile broadband, is being fast-tracked as part of 3GPP Release 15; and the 5G standalone new-radio specification will be in Release 16.
With non-standalone radio, the 4G LTE band provides an "˜anchor' for carrying control and signalling information, while the 5G band acts as a data pipe. In contrast, with 5G standalone radio, the new 5G network architecture is used for both the control plane and the user plane, which carries the data.
Thanks to the development of the non-standalone radio, early delivery of 5G enhanced mobile broadband is more economically viable for network operators. That's because these operators can leverage the existing 4G LTE modems and transceivers in devices and infrastructure along with the existing evolved packet core. Or, to put it another way, operators can deliver non-standalone radio by enhancing existing 4G LTE infrastructure, rather than complete building out of next gen core, standalone radio 5G networks. It is this approach that is enabling large-scale 5G, enhanced mobile broadband trials and deployments for as soon as 2019, rather than the 2020 timeline, previously slated.
Initially, the deployment of enhanced mobile broadband is expected to focus on frequencies below 6 GHz. That's because this frequency domain supports broader coverage, and it is better suited than millimetre-wave frequencies for the support of network connections in mobile devices. Helping the transition is the allocation of new global frequency bands "“ 3.3 GHz and 5 GHz have already been allocated or are under consideration, due to near-global availability. These efforts should lead to the re-farming of TDD-LTE bands, such as 42 and 43, that will also be used as 4G anchor bands in different regions; and the introduction of new bands n77, n78 and n79. The latter three will be the first 5G bands that are allocated solely for 5G data transmission.
RF front-end challenges
Equipping handsets with 5G non-standalone radio and complex 4G LTE Advanced CA functionality will be a challenge for both device and chipset makers. Their efforts will be directed at producing non-standalone, RF front-ends that support 4G and 5G requirements in a single module, and enable global coverage, as this minimizes the need for handset localization. These requirements will create an unprecedented set of technical challenges for the RF front-end, especially on the transmit side. Challenges will include the delivery of high-power efficiency and linearity for 4G and 5G waveforms, while supporting very wide bandwidth and delivering high output power, including the new Power Class 2 standard. Power Class 2, or HPUE, has recently been adopted as a method to improve coverage for handsets, but increasing the power by 3dB over the traditional Power Class 3 mobile device standard.
These various standards are now well underway, with two 5G uplink waveforms moving toward formal approval within 3GPP: Cyclic Prefix Orthogonal Frequency Division Multiplexing (CP-OFDM) and Discrete Fourier Transform-spread-OFDM (DFT-s-OFDM). Front-ends for 5G, including non-standalone radio, will have to support both of these waveforms. Of these two, CP-OFDM presents the greatest challenges, but has the most promise, offering several key advantages for network operators. Its attributes include providing the most efficient spectral packing of resource blocks (it is up to 98 percent), and offering benefits in spatial multiplexing, so that it can support multiple input, multiple output (MIMO) technologies. Thanks to these merits, CP-OFDM is the leading candidate when the priority is to maximize network capacity, such as in cities and within buildings. The challenge is that CP-OFDM generates much higher peak-to-average power ratios than 4G LTE. The implications are the need for greater power back-off in the power amplifier and high linearity over a wide range of power levels.
The alternative, DFT-s-OFDM, provides less-efficient spectral packing "“ it is up to 90 percent "“ and it is less effective for MIMO. However, this form of 5G uplink, which has the same waveform as the LTE uplink in use today, offers a greater operating range, thanks to lower peak-to-average power ratios. Due to these characteristics, one would expect the use of DFT-s-OFDM when the priority is to maximize coverage, such as in less densely populated areas.
Massive bandwidth
One of the big differences between 4G LTE and 5G is the bandwidth of the signals: it will be typically 100 MHz per carrier, compared with up to 20 MHz per carrier with LTE (although up to 60 MHz has been supported using CA). The combination of the wider bandwidth and the new 5G waveforms propels peak-to-average power ratios to far higher levels than they are in any preceding cellular communications standard. Consequently, the RF front-end needs to maintain sufficient gain and high linearity across very wide channels within high-frequency bands.
There are power efficiency challenges associated with the wider-bandwidth signals. To maximize efficiency for 4G LTE, common approaches are to introduce envelope tracking and digital pre-distortion. If envelope tracking is adopted, efficiency is optimized by continuously adjusting the supply voltage of the power amplifier, so that it tracks the RF envelope.
Unfortunately, today's envelope trackers can only support a bandwidth of up to 40 MHz. Due to this limitation, envelope tracking is unsuitable for 100 MHz 5G signals. Instead, the power amplifier has to be operated in average power tracking mode (with a fixed voltage), while providing high linearity operation across the entire frequency range. The upshot is a substantial fall in the efficiency for the transmit chain, along with a very challenging high linear power requirement for the power amplifier design.
As is the case for LTE, 5G requires a multi-gain state PA with relatively quick gain settling times. If the most complex downlink modulation scheme is adopted, 256QAM, high linearity is required in the low-noise amplifier as well. Key figures of merit are a superior error vector magnitude, and an improved second-order intercept point over that needed for 64QAM LTE signals of today.
Higher output powers
To maximize the operating range of the handset, efforts are being directed at increasing the RF output power, as this can compensate for greater propagation losses at high frequencies. This requires support for the 3GPP Power Class 2 standard, which doubles handset output power at the antenna "“ it increases from 23 dB, the previous standard, to 26 dB. By turning to Power Class 2, operators are able to use high-frequency bands to realise an operating range comparable with that provided by lower-frequency bands, without the need to build extensive new wireless infrastructure.
Figure 1. 5G application categories.
That's not the only benefit, however. Power Class 2 also improves in-building reception. In contrast to 4G LTE, where the 3GPP Power Class 2 standard is only utilized in a limited set of TDD-LTE bands, with 5G, Power Class 2 is a baseline requirement across all new bands of operation. Consequently, all 5G RF front-ends will have to support this high output power, while managing the associated thermal challenges, including heat dissipation.
On top of these thermal challenges, the move to 5G non-standalone radio will give device manufacturers the tricky task of having to cram even more complex RF content into the already crowded space allocated to the RF front-end. Unlike 4G LTE, where MIMO is optional, 5G implementations are expected to use 4 x 4 MIMO in the downlink as per the standard; some devices may use 2 x MIMO for the uplink as well, providing an unprecedented 200 MHz of uplink bandwidth for extremely high data rates. Accommodating the additional RF chains within the handset will require highly integrated modules. In some cases, additional antennae may need to be introduced in some handsets to support the high-frequency bands, leading to further size and RF isolation challenges.
RF front-end design
Engineers must take great care when designing the RF front-end, so that they can fulfill the extraordinary set of efficiency, bandwidth, linearity, and output power requirements demanded by 5G non-standalone radio. Meeting the overall requirements hinges on optimization of the performance of key components within the RF front-end, such as the power amplifier, low-noise amplifier and filter.
The key to success is to combine class-leading components, manufactured with different process technologies. Using advanced packaging techniques, these components can be united into integrated modules that save space, increase power efficiency and improve thermal performance.
At Qorvo, we have designed an RF front-end that meets the global requirements of 5G non-standalone radio (see Figure 2). This front-end, our QM19000 that was unveiled at this year's Mobile World Congress, features a filter with a very wide passband to support the allocated 4G and 5G bands "“ it supports a 900 MHz range, spanning 3.3 GHz to 4.2 GHz.
One attribute that this filter does not have to have is "˜steep skirts', which are needed in other designs to avoid interference in lower-frequency regions of the spectrum that are more congested. Thanks to this, it is not necessary to use bulk acoustic wave filter technology, although this could be used as requirements evolve in future.
Figure 2. The Qorvo QM19000, a 5G non-standalone radio mobile RF front-end.
For the low-noise amplifier, a variety of technologies are available, including silicon-on-insulator, but the GaAs pHEMT has been the ideal choice, as it offers advantages in meeting the strict linearity requirements.
For meeting 5G non-standalone radio requirements, the power amplifier is critical. It has to handle both 4G and 5G transmissions. In the first implementations, envelope tracking will be used for 4G LTE to maximize overall system efficiency. However, this technology is unsuitable for 5G, due to the much greater bandwidth, typically 100 MHz. Due to this, the power amplifier has to support multiple power management schemes: envelope tracking for 4G, and average-power tracking for 5G. As it must meet performance requirements in both modes, it needs to combine high saturated efficiency in envelope-tracking mode with linear efficiency in average-power tracking mode. This amplifier must also meet linearity and gain requirements when supporting both 4G and 5G transmissions. That includes when it is operating with the power back-off, a necessary condition for CP-OFDM, due to the high peak-to-average power ratio.
To meet all the requirements for 4G and 5G, including saturated and linear efficiency, it is necessary to use a power amplifier manufactured with a compound semiconductor technology, such as our HBT5 GaAs process. Successive generations of this process have delivered increased gain and power output to meet new requirements, such as Power Class 2, while improving efficiency. Power amplifiers produced with our HBT5 process offer high thermal performance, with excellent heat dissipation characteristics, thanks to the use of our copper-bump packaging technology. Even with a two-stage power amplifier built with our HBT5 process, additional amplification may be required to meet requirements for higher output power, such as when supporting Power Class 2 and during use of CP-OFMD waveforms (where transceiver drive levels are anticipated to decrease by up to 3 dB). To meet these needs, our QM19000 includes an additional variable gain amplifier. This product also features sophisticated power management, for switching between envelope-tracking mode and average-power tracking mode.
Additional merits of the QM19000 include high saturated power-added efficiency, while also maintaining good linear efficiency across a wide range of positive supply voltages. The module maintains efficiency across the entire 3.3-4.2 GHz frequency range, with the use of GaAs, rather than SiGe, providing higher gain than other technologies at these high frequencies. Consistent gain is provided across the supported frequency range when transmitting a 100 MHz CP-OFDM signal, while maintaining high linearity.
It is clear that establishing a non-standalone radio standard for mobile broadband has enabled operators to accelerate plans for 5G. At the same time, it has created challenging new mobile RF front-end requirements, including the need to support both 5G and 4G waveforms and massive bandwidth while providing high gain, efficiency and linearity under a wide range of conditions. Integrated RF front-ends, including high-performance PAs based on compound semiconductor technology, are key to solving these challenges. As the other 5G application areas advance toward deployment, they will generate other RF challenges, requiring innovative high-performance solutions for devices and wireless infrastructure. These solutions are likely to leverage the unique performance characteristics of compound semiconductors