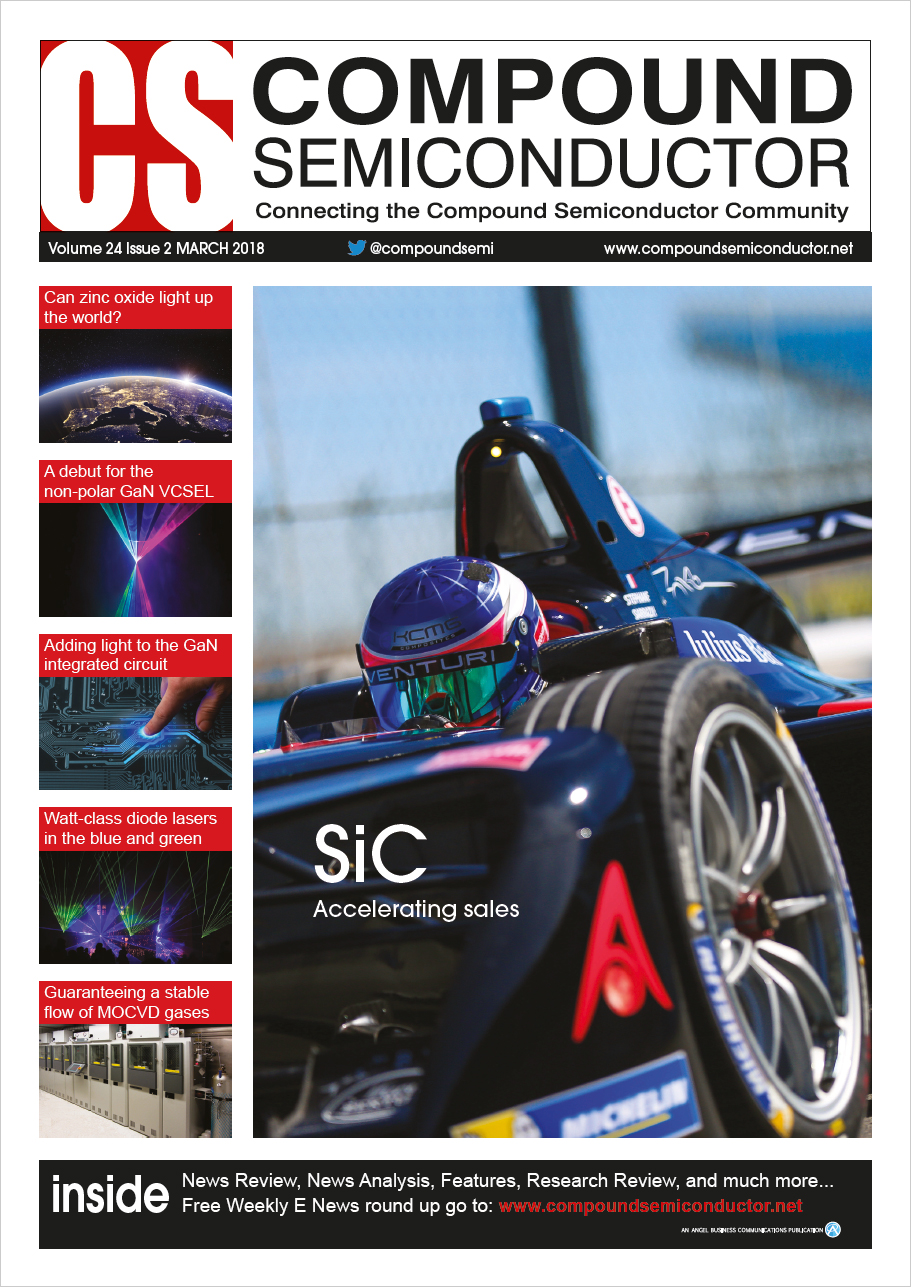
The world's first CW non-polar GaN VCSEL
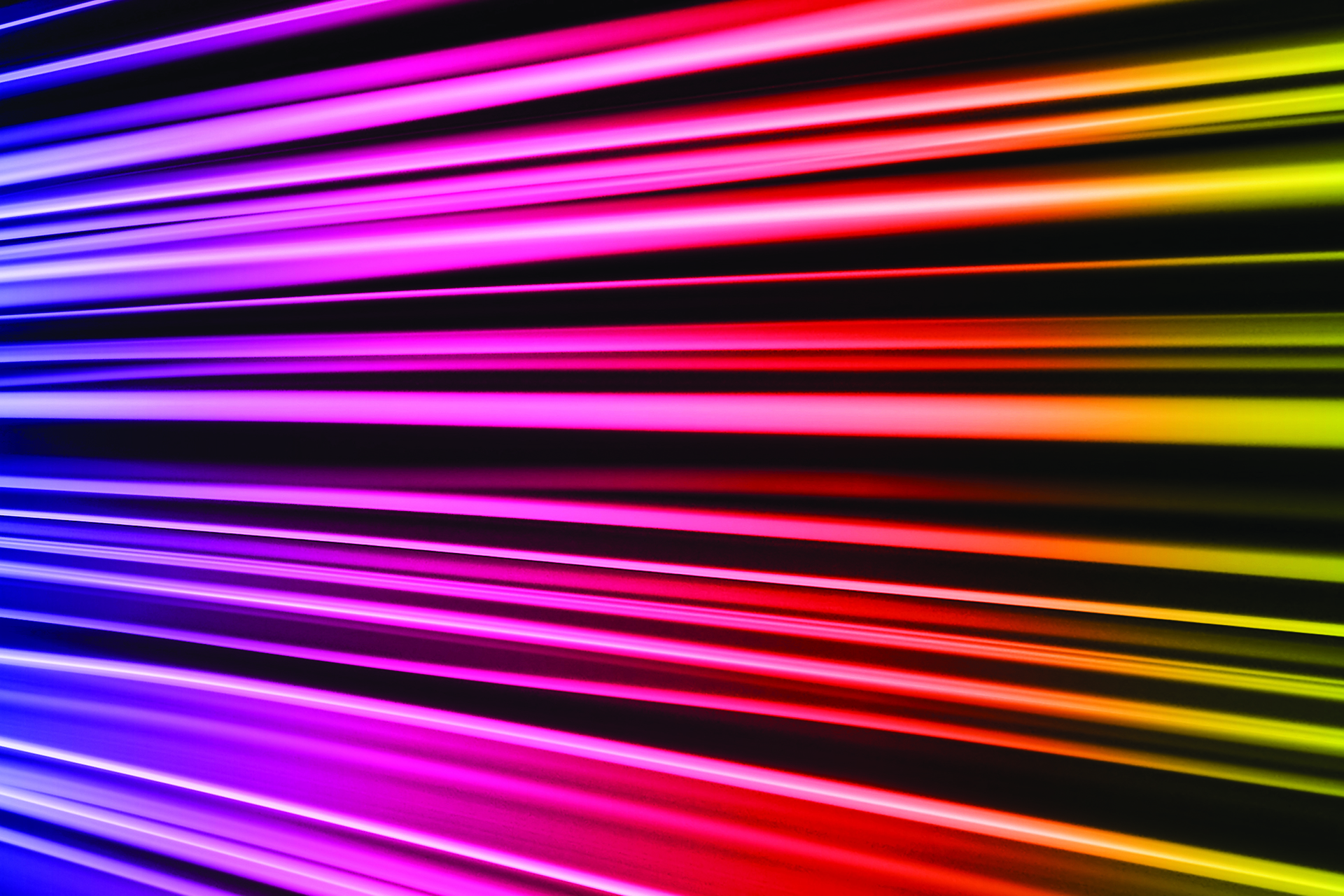
Polarization-locked arrays of violet, blue and green lasers are now on the horizon, thanks to the first demonstration of non-polar GaN VCSELs delivering continuous-wave operation by Charles Forman, Steven Denbaars and Shuji Nakamura from the solid state lighting and Energy Electronics Center at University of California, Santa Barbara
The VCSEL has many desirable attributes. Sporting a far smaller size than its edge-emitting cousins (see Figure 1), it is capable of higher modulation speeds for data communication, and lower power consumption, which is ideal for battery-powered devices. Its only significant drawback is its relatively low output power, but this can be overcome by arranging these devices in densely-packed, two-dimensional arrays.
VCSEL production is now well established. Leading the way are infrared VCSELs based on the GaAs family, which have been capable of CW operation since the late 1980s and were commercialised in the 1990s. These devices have replaced edge-emitters in computer mice and laser printers, and are now being deployed in mobile phones. In the latter application, Finisar is enjoying tremendous success, having just been awarded $390 million to increase its R&D and production of VCSELs, which are key components in the iPhone X TrueDepth camera and AirPod proximity sensor.
Finisar's success could be just the tip of the iceberg for VCSEL manufacturers. Today, this market is limited to red and infrared emission, using GaAs and InP-based VCSELs. But if the spectral domain could expand to encompass blue, green and shortwavelength sources, VCSELs could start to penetrate a whole world of untapped applications in display, illumination, and sensing technology.
Combining red VCSELs with those emitting in the green and blue could create full-colour light engines for displays and projectors, while the low power of all these sources makes them ideal for portable electronics, such as wearable displays. In addition, VCSELs could be deployed in laser-based lighting, and in Li-Fi, where they could provide even faster modulation speeds than edge-emitters, which are already hundreds of times faster than LEDs, enabling a hike in data transfer rates.
At the Nakamura Lab at the University of California, Santa Barbara, we are taking important strides in this direction: we have demonstrated the world's first non-polar GaN-based VCSEL that is capable of lasing under continuous-wave (CW) operation. This is a significant breakthrough because non-polar VCSELs offer many advantages over their polar cousins, including: an absence of polarization-related electric fields in the active region that can improve radiative efficiency; and anisotropic gain, which enables the fabrication of arrays that provide a fully polarized emission source. The latter attribute increases the opportunities for the GaN VCSEL, by opening up applications ranging from optical sensing to increased data transmission rates via polarization division multiplexing.
GaN VCSEL progress
Progress in GaN VCSELs has not been easy. 12 years elapsed between Nakamura's report of the first blue laser in 1996 and the unveiling of the first electrically pumped GaN VCSEL, by Tien-Chang Lu and colleagues from National Chiao Tung University (NCTU). Even a decade on from that first great success, just eight research groups have successfully demonstrated an electrically-injected, GaN-based VCSEL.
Figure 1. Violet GaN VCSEL (left) and blue edge-emitting laser (right) under electrical injection. In comparison to edge-emitters, VCSELs have many merits: orders of magnitude smaller active volumes, lower thresholds for lasing with lower power consumption, high-speed modulation, 2D arraying capability, onwafer testing, circular output beam with low divergence, higher spectral purity, and ability for single-longitudinal mode operation.
Understand the difficulties associated with making a GaN VCSEL, and it is easy to appreciate this apparent lack of progress. Due to an extremely short gain path length of typically 10-30 nm, this class of laser requires a pair of mirrors with a reflectivity in excess of 99 percent. It's a requirement that is relatively easy to fulfil for GaAs VCSELs, wherein mirrors can be formed from alternating, lattice-matched layers of doped, quarterwavelength-thick GaAs and AlGaAs "“ a pairing that creates electrically conductive epitaxial distributed Bragg reflectors (DBRs). However, with a GaN-based VCSEL, fully epitaxial DBRs have not been an option. There have been several growth challenges, along with difficulty activating p-GaN that is buried below epitaxial layers.
Figure 2. Focused ion-beam cross-sectional scanning electron microscopy revealed problems with earlier GaN VCSELs. The thermally insulating bottom DBR forces heat to flow through a thin gold path toward the flip-chip substrate The thermal performance is further impaired by cracks in this thin metal that form during the Au-Au flip-chip bond.
Instead, teams have produced VCSELs with either two sets of dielectric DBRs or a hybrid design with a bottom epitaxial DBR; however, even the growth of just one epitaxial DBR is challenging, due to a lattice mismatch between AlGaN and GaN that leads to cracking.
One team that has had success with the latter approach is that of Tetsuya Takeuchi and co-workers at Meijo University. Using lattice-matched, n-type conducting layers of AlInN and GaN, they have made a mirror with the required level of reflectivity. However, this requires 46 pairs of AlInN and GaN, due to the low index contrast. Consequently, it's a lengthy growth process, demanding precise thickness control to match the peak reflectance with the lasing wavelength.
Figure 3. The dual-dielectric DBR GaN VCSEL with an ion implanted current aperture and tunnel junction intracavity contact, which reduces internal losses compared to ITO. The structure prior to the flip-chip bond is shown on the left. Photoelectrochemical etching of a sacrificial multiple quantum well enables precise cavity length control when removing the m-plane GaN substrate.
The more common approach is to sandwich the active region between two sets of dielectric DBR mirrors. Typical combinations, such as the pairing of SiO2 and Ta2O5, are relatively easy to deposit using e-beam deposition or sputtering. What's more, thanks to higher index contrast, this class of DBR requires a relatively smaller number of mirror pairs "“ and it has a much wider high-reflectivity stopband, easing alignment with the lasing wavelength.
However, it's not all smooth sailing with a dual-dielectric DBR design. Mirrors have to be deposited on both sides of the device, within a few microns of the active region. One way to do this, adopted by Tatsushi Hamaguchi and researchers at Sony, is epitaxial lateral overgrowth on top of a previously-deposited dielectric DBR on GaN. VCSELs produced in this manner, which feature an ion implanted aperture, can produce more than 1 mW, which is one of the highest reported peak output powers for this class of device. However, although epitaxial lateral overgrowth is promising, it is a sophisticated technique with several growth challenges.
An alternative approach, avoiding lateral overgrowth, is the creation of dual-dielectric DBRs with a flip-chip design. In this case, the price to pay is an increase in the complexity of the fabrication steps, including the need for flip-chip bonding to a submount and the removal of the growth substrate to access the n-side of the device. Conventional polishing and thinning is one option for removing the growth substrate. However, controlling VCSEL cavity length is challenging "“ and this is critical to device performance, because it impacts the electromagnetic standing wave field within the cavity.
We take a different tack to remove the growth substrate, using photoelectrochemical etching to selectively etch a sacrificial multi-quantum well. This enables precise control over cavity layer thicknesses and ultimately allows us to accomplish a key goal in VCSEL design: maximizing the gain enhancement factor. Success results from aligning the active region with the optical field standing-wave peak, which increases the confinement factor and helps decrease the threshold for lasing. Even higher levels of performance are possible by trimming optical absorption. To realise this, layers with higher levels of optical loss, such as ITO and highly-doped layers, have to be aligned with standing-wave nulls.
Figure 4. Summary of nonpolar m-plane GaN VCSEL fabrication. While c-plane GaN VCSELs are polarized in random directions, m-plane GaN VCSELs are consistently polarized in the a-direction, leading to VCSEL arrays with a 100 percent polarization ratio.
There are additional challenges associated with dielectric DBRs, which stem from their electrically and thermally insulating nature. As current injection is not possible through non-conductive DBRs, carriers need to be injected from the edges of the aperture, and the device needs to be designed so that current can spread toward the centre of the cavity. However, uniform current injection is not easy as p-type GaN has a notoriously low conductivity "“ typically around 1 Ω-1 cm-1 "“ inhibiting lateral current injection.
One of our innovations is to inject carriers into the device with an MBE-grown, relatively transparent tunnel junction and current spreading layer. This is superior to the more common approach of employing an intracavity, current-spreading layer made from ITO. That's because this oxide is flawed: it is not completely transparent at blue and violet wavelengths, and it degrades VCSEL performance due to significant optical loss, even when ITO is placed at the null of the optical standing-wave.
A non-polar design
In addition to the promise of higher radiative efficiency, which we championed earlier in this article, nonpolar GaN VCSELs offer the opportunity to employ thicker wells without reduced radiative efficiency, and anisotropic gain that leads to a 100 percent polarization ratio for m-plane VCSELs. While individual c-plane VCSELs are polarized in random directions, m-plane VCSELs are consistently polarized along the a-direction, opening the door to 100 percent polarization-locked VCSEL arrays.
Another crucial advantage of the non-polar m-plane of GaN is that it is compatible with our photoelectrochemical etching technique. During this process, ultraviolet light creates photogenerated holes in the sacrificial wells that oxidize the surface and dissolve in a potassium hydroxide solution. Note that our etching technique cannot be applied to c-plane VCSELs because potassium hydroxide roughens the nitrogen-face GaN "“ this is not acceptable, because it would cause severe scattering loss on the n-side.
Significant cost savings may also result from our etching process. Bulk GaN substrates are pricey, but because they are removed from the device, it is possible that they could be reused for the next batch of VCSELs.
Figure 5. Focused ion-beam cross-sectional scanning electron microscopy image of a GaN VCSEL utilizing Au-In bonding to create a much thicker metal path for heat flow. This scheme for superior thermal management led to the first demonstration of CW operation for non-polar GaN VCSELs.
Our first milestone in non-polar VCSELs came in 2012, when Casey Holder demonstrated a device with a dielectric current aperture and an ITO intracavity contact. Refinements followed, led by John Leonard, who introduced three new GaN VCSEL designs, featuring ion implanted current apertures, tunnel junction intracavity contacts (replacing ITO), and photoelectrochemically etched air-gap apertures. Ion implantation produced the best results for current confinement, and has been adopted by Sony, featuring in devices reported in 2016.
From this era, our most impressive performance came from a VCSEL with an aluminium-ion-implanted current aperture and an MBE-grown, GaN-based tunnel junction intracavity contact. This device delivered a pulsed peak output power of 0.5 mW, but could not lase under CW operation.
Figure 6. Current, light and voltage characteristics of a 6 μm aperture diameter VCSEL with a 23 λ cavity length (a) under pulsed operation and (b) under CW operation. This is the first demonstration of CW operation for nonpolar GaN VCSELs. CW lasing was stable for over 20 minutes of continuous testing. The threshold current was 12 mA under pulsed operation and 10 mA under CW operation.
Failure to provide CW operation stems from a bottomside dielectric DBR that is thermally-insulating, and thus inhibits downward heat flow. Heat is extracted from the device by flowing it around the bottom DBR through a thin gold contact that leads to the flipchip substrate. Unfortunately, the heat transferring capabilities of this gold contact are compromised by cracks and voids within it "“ they are exposed by scanning electron microscopy of cross-sections, prepared by a focused ion-beam (see Figure 2).
The origin of these imperfections is the hightemperature, high-pressure process used to unite gold-coated surfaces by thermocompression, flip-chip bonding. Damage extends to the devices, which have low yield and are plagued by cracks. To address this, we bond gold to indium, to create the first non-polar VCSELs that are capable of CW operation.
Making VCSELs
We produce our latest generation of VCSELs (see Figure 3) by taking m-plane GaN substrates with an intentional -1° miscut in the c-direction, loading them into an MOCVD chamber, and growing a 1.2 μm-thick n-GaN template, followed by a sacrificial multiple quantum well, 26 nm of n++ GaN, 15 nm of n-AlGaN, 3.2 μm of n-GaN, an active region with two 14 nm-thick InGaN quantum wells separated by a 1 nm-thick GaN barrier, 5nm of p-AlGaN, 60nm of p-GaN, and 14nm of p++GaN.
After dry etching past the active region to create a mesa, we use aluminium ion implantation to form the current aperture, before turning to Erin Young's expertise with ammonia MBE to grow an n-GaN tunnel junction and current spreading layer. This involves depositing a 40 nm-thick layer of n++GaN, followed by 62 nm of n-GaN and 40 nm n++GaN. To help smoothen the surface and ultimately reduce VCSEL scattering loss, we add a non-incorporating indium surfactant to the growth process. SiN is then deposited, so that the sidewall of the active region is protected during subsequent photoelectrochemical etching.
The next steps are the addition of a 16-period SiO2/Ta2 O5 DBR, followed by a deeper dry etch to expose the sacrificial multiple quantum well. After this, a Ti/Au p-contact metal is deposited conformally around the DBR, before this structure is flip-chip bonded to a sapphire substrate coated with an indium-rich alloy of indium and gold. Fabrication of the VCSEL is completed (see Figure 4) by using a potassium hydroxide solution, alongside 390 nm excitation from an LED array, to photoelectrochemically etch the sacrificial multiple quantum well, before adding a Ti/Au n-electrode, followed by a 12-period SiO2/Ta2 O5 DBR. Note that a rough residue forms after photoelectrochemical etching, which is removed by swabbing in Tergitol detergent.
Combining a relatively thick, indium-rich goldindium alloy on the flip-chip substrate with gold atop the device on the GaN substrate produces a big improvement in thermal performance "“ and ultimately enables CW operation. The gold-indium system is ideal for flip-chip bonding, because it has a unique low-temperature liquid phase that occurs above 156 °C for gold-indium alloys with an indium content of more than 54 percent, in terms of weight. This enables flip-chip bonding at much lower temperatures and pressures than bonding between gold surfaces, leading to improved yield with significantly fewer cracked devices. While previous devices had a thin metal pathway for heat transport, gold-indium bonding completely embedded the bottom-side DBR in metal (see Figure 5), greatly improving thermal performance, according to COMSOL simulations.
Last, but not least, the thermal capabilities of the device are further improved by increasing the GaN cavity length from 7 λ to 23 λ. This lowers the active region temperature as heat can spread throughout a thicker thermally-conductive GaN cavity.
CW operation
We have produced VCSELs with 6 μm and 8 μm aperture diameters that deliver CW operation with stable lasing for more than 20 minutes. For the device with the 6 μm aperture, peak output power is 150 μW under CW operation, and 700 μW under pulsed operation (see Figure 6). Meanwhile, for a variant with a 10 μm aperture, peak output can exceed 1 mW, which is approximately double that from previous non-polar GaN VCSELs. We attribute this superiority to a longer cavity length, which aids mode alignment at higher current densities, thanks to a reduction in the longitudinal mode spacing.
The emission wavelength red-shifts with drive current. The peak in spontaneous emission occurs at 403 nm, and lasing initially appears at 406 nm and 412 nm under pulsed operation above the threshold current of 12 mA. Even when driven in pulsed mode, the peak gain red-shifts at higher currents, due to heating, with a dominating 419 nm lasing mode emerging above 33 mA. As this longer-wavelength mode appears, differential efficiency increases from 0.3 percent to 0.8 percent. We attribute this to lower absorption, a reduction in scattering loss, and lower mirror reflectivity at longer wavelengths.
Stronger lasing at longer wavelengths also results in an interesting phenomenon: the threshold current uncharacteristically decreases to around 10 mA under CW operation, due to the higher temperature redshifting the peak gain, resulting in longer wavelength modes emerging at lower currents. An example of this is that the high-intensity 419 nm mode appears above 33 mA under pulsed operation, while the 420 nm mode appears much earlier, at 15.1 mA, under CW operation (see Figure 7).
This red-shifting of the gain spectra offers an insight into the VCSEL operating temperature. Measuring the red-shift in peak spontaneous emission under CW operation suggests a VCSEL operating temperature of 163 °C at 15 mA and a thermal impedance of 1400 °C/W. Both these values compare favourably with thermal simulations.
Figure 7. Emission spectrum at various current injection levels for a 6 μm aperture diameter VCSEL with a 23λ cavity length under CW operation. Under pulsed operation, lasing modes appeared at 406 nm, 412 nm, and 419 nm. Under CW operation, the redshifted peak gain resulted in only two lasing wavelengths at 413 nm and 420 nm. The peak gain redshifted with increasing current and longerwavelength modes increased while shorter-wavelength modes decreased in intensity.
Close inspection of the emission from the device reveals that lasing is centred within the aperture, appearing as the fundamental transverse mode above threshold (see Figure 1). This is a significant improvement compared with previous m-plane VCSELs, which typically exhibit filamentary lasing "“ that is, random lasing spots appearing in the aperture. We are still in the process of confirming the reasoning behind this improvement. It may be related to the removal of a rough oxide layer, generated during photoelectrochemical etching.
Our work is in its infancy, and we are sure that non-polar VCSELs can deliver far higher levels of performance. Refinements could include switching from sapphire for the flip-chip substrate to a more thermally conductive material, such as copper or SiC. An increase in output power should follow, allowing the device to take another step towards commercialisation, and the promise of significant sales in many markets.