Improving modeling of high-efficiency solar cells
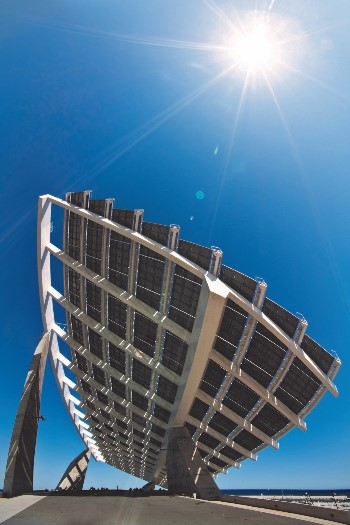
Analytical models accounting for reflections and photon recycling provide accurate predictions of device results.
BY MATTHEW LUMB FROM THE GEORGE WASHINGTON UNIVERSITY AND THE US NAVAL RESEARCH LABORATORY, ROBERT WALTERS FROM THE US NAVAL RESEARCH LABORATORY AND MYLES STEINER AND JOHN GEISZ FROM THE NATIONAL RENEWABLE ENERGY LABORATORY.
Many researchers in the solar sector are pursuing the same goal "“ to come up with a technology that increases photovoltaic efficiency. It's a worthwhile aim, because if a cell can extract more electrical energy from the sun's rays, solar power may become more affordable, leading to increased sales and better economies of scale.
Several approaches are available for developing higher efficiency cells. One option is to design and evaluate a new architecture in the lab, and an alternative approach is to model new device designs to see if they are superior. In practice, a mix of both these approaches tends to work best, with measurements on real devices verifying the capability of a new cell design that produces promising results in modeling efforts.
Whatever materials are used to build a conventional solar cell, ultimately efficiency is limited by a fundamental process: radiative recombination. It follows from the reciprocity of absorption and emission processes that if all other loss mechanisms are entirely suppressed, the resulting, perfect solar cell would also, in fact, be a perfect LED.
This relationship between a faultless LED and a solar cell has been known for many, many years, and in the 1950s it was employed by Nobel-prize-winning physicist William Shockley and co-worker Hans-Joachim Queisser to derive the efficiency limit for a solar cell. This model, which accounts only for band-to-band radiative recombination of electron-hole pairs, assumes that all other processes are ideal. It is widely used by researchers in the photovoltaics community today, and one of its strengths is that it is able to express the potential of competing technologies in the simplest terms. However, real world solar cells are seldom close to ideal. Efficiency is impaired by optical losses, non-radiative recombination and electrical losses, which usually combine to make the Shockley-Queisser limit a gross overestimation.
Models that can provide a more realistic value for efficiency are highly desired, because they can aid efforts to improve solar cells. This need is particularly acute for III-V cells, which come closer than many other material systems to reaching their fundamental limit, thanks to advances in epitaxy, processing and optical management. In space, these cells are united with those made from germanium to power satellites, and in sunny climes these cells lie at the heart of power generation systems featuring mirrors or lenses that focus the sun's radiation by factors of several hundred or more.
In the 1970s, Harold Hovel and Jerry Woodall introduced a more sophisticated model that is better at capturing the performance of single-junction cells.This pair of researchers, who were working at IBM Research Laboratory in Yorktown Heights, New York, developed an analytical drift-diffusion model that contains all the real world losses (see "The Hovel and Woodall model" for details).
However, this approach is far from perfect, because it fails to account for the contribution to energy generation that occurs in cells employing a high reflectivity back mirror, which increases absorption within the cell and enhances photon recycling. A more complete model would include coherent and incoherent optical effects, such as contributions from back surface reflectors, which increase the probability of photon absorption; and it would account for photon recycling, a process where photons spontaneously emitted by the material through radiative recombination are reabsorbed by it.
A GaInP cell that is luminescing under blue light, a result of substantial photon recycling enhancement due to the back reflector.
Previously, numerical methods have been employed to construct a fully numerical drift-diffusion model that accounts for all these effects. Including them all is essential for accurate modeling of today's best devices, which are coming very close to the fundamental efficiency limit.
Strengths of these numerical models include their flexibility and precision. But these merits have to be weighed against several downsides, which can be addressed by turning to an analytical model that includes the additional optical effects "“ which is an approach that has been pioneered by our team from the US Naval Research Laboratory, George Washington University and the National Renewable Energy Laboratory (NREL).
One of the virtues of our analytical model is that it avoids the need for advanced numerical techniques for solving boundary value problems on a mesh grid, resulting in a model that is easy to develop. What's more, the analytical model provides an excellent intuitive understanding of the inner workings of a solar cell, due to separate treatment of different regions within the device. All this is possible while delivering comparable accuracy to the numerical model, so long as we can meet the assumptions upon which the model is based.
To date, our work has been restricted to the modeling of single-junction devices. However, it should be possible to extend this effort to multi-junction variants. This will require modification of the model to include luminescent coupling, which occurs when the emission from the top cell generates photons in the cell beneath.
Handling multiple reflections"¦
To account for the multiple reflections inside the cell when a back mirror is present, we have added a generation function to the drift-diffusion model. It is possible to determine a compact analytical expression for this function at every point in the solar cell by summing the fields of the multiple reflected beams in the absorbing region. This expression can then be incorporated into the drift-diffusion calculations, and solved in an analogous way to the original Hovel and Woodall model. Note that this approach can be applied to both coherent and incoherent reflections from the back surface reflector.
We can illustrate this approach by considering the simple case of a GaAs absorber with a coherent gold back reflector (see Figure 1). At short wavelengths GaAs is strongly absorbing, so the generation function resembles that of Beer's law "“ this states that as light passes through a substance, its intensity decreases exponentially. For wavelengths near the bandgap of GaAs, this law is not valid, and the generation function deviates significantly as GaAs becomes semi-transparent. In this wavelength region, the solar cell acts like a Fabry-Perot cavity, with the forward and reverse propagating fields interfering to produce an overall enhancement in the photon density absorbed by the GaAs. This leads to an increase in photocurrent.
Figure 1: A generation function accounts for optical effects in a cell. A modified form of this function is used when a gold mirror is added to the cell's backside to create a coherent back-surface reflector (BSR).
Adding a gold mirror to the thin GaAs homojunction solar cell leads to a significant increase in its external quantum efficiency (see Figure 2, which shows that modeling can replicate experimental measurements by capturing coherent optical effects arising due to the back surface reflector). However, these demonstration devices were fabricated without a front side anti-reflection coating, leading to losses of approximately 30 percent at the front surface. Therefore, the mirrored cell in this example has not reached the realms of ultra-high efficiency.
Figure 2: Modeled and experimental values of the external quantum efficiency of two GaAs solar cells of the same 1 mm thickness: structure 1 has no back reflector, whereas structure 2 has a high reflectivity gold back reflector. Note that the external quantum efficiency is a measure of the spectrally resolved probability of an incident photon being captured and converted into an electron in an external circuit.
Production of these cells begins with MOCVD growth of the epitaxial layers on a GaAs substrate. During post-growth processing, if an external back metal contact is deposited on the rear side of the substrate, the thick absorbing substrate will act as a photon sink to any internally emitted radiation (this is the case for structure 1 in Figure 2).
It is possible to insert a highly reflective back contact by removing the substrate and depositing the mirror directly on the rear side of the epitaxial layers. An innovative way to do this, pioneered at NREL in 2007, begins with the growth of the solar cell in an inverted configuration, with the front layers grown first and the back layers grown last. Layers are then re-orientated during post-growth processing to create a structure where the rear surface of the actual solar cell is immediately accessible. A highly reflective metal or dielectric-metal mirror can then be deposited on the growth surface (this is the case for structure 2 in Figure 2, and the device that results from this approach is shown in Figure 3).
Figure 3: An inverted growth process enables a mirror to be positioned directly beneath the cell, enhancing light absorption. Another benefit of this approach is that it allows re-use of the substrate.
Fabrication of this type of cell is completed by bonding the epistructure to a handle wafer, such as silicon or a flexible plastic, and then removing the native substrate "“ wet chemical etching is one way to do this, though other techniques have been developed that allow the substrate to be preserved and reused. Epilayers are then processed into individual solar cell devices with front contacts.
"¦ and photon recycling
In addition to multiple reflections, the other significant improvement of our analytical model over that developed by Hovel and Woodall is the inclusion of photon recycling. In a perfect cell, all of the solar radiation that impinges on the front surface will be absorbed. Then, in order to generate power from the device, some radiative recombination must occur. This is an inescapable loss, but in an ideal cell all the non-radiative processes are negligible, and all of photons generated by radiative recombination can only escape the device through its front surface.
When a cell is generating power, there are actually a great number of recombination events happening simultaneously, with photons emitted in all directions inside the absorber. Ideally, most of those photons are recycled into new electron-hole pairs (see Figure 4).
Figure 4: If photon recycling is ideal, the only photons that are able to escape the absorber pass through the front surface; all other photons are recycled to generate new electron-hole pairs. The inclusion of a back surface reflector improves the probability that incident sunlight is absorbed within the device.
Progress towards this goal is possible with a back surface reflector, which suppresses photon escape through the rear surface of the cell and thus improves the external radiative efficiency.
One of the consequences of photon recycling is a cut in the net rate of radiative recombination. This can also be stated in another way − as photon-recycling increases, the radiative lifetime of minority carriers increases until the external radiative efficiency limit is reached. However, if photon recycling is to deliver a performance improvement, it is paramount that the internal radiative efficiency of the material "“ that is, the fraction of recombination events that are radiative − approaches unity. When this happens, the net lifetime of minority carriers increases due to photon recycling, which leads to an increase in their diffusion length.
In III-Vs cell made today, material quality is exceptionally high, enabling cells to operate at very high internal efficiencies. In these devices, the voltage produced by a solar cell provides an excellent indicator of photon recycling, which impacts the recombination rate and therefore the dark current.
Our efforts at capturing photon recycling in our model (see "Including photon recycling in an analytical model") have helped us to appreciate the importance of maximizing the external radiative efficiency of solar cells. Motivated by this, members of our team at NREL have developed an important modification to conventional homojunction solar cells: the thick emitter concept.
The traditional architecture for a high efficiency III-V cell includes a thin, highly doped n-type (emitter) layer atop a thick, lower doped p-type (base) layer. Short wavelength light, with a short penetration depth, is predominantly absorbed in the emitter layer and longer wavelength light in the base layer. In contrast, our new design features a thick, moderately doped n-type emitter layer atop a thin p-type base layer, giving rise to a high external radiative efficiency.
There are several reasons why a thickening of the emitter boosts performance. First, so long as the diffusion length of minority carriers significantly exceeds the thickness of the quasi-neutral regions (which is required for efficient minority carrier extraction in solar cells), a structure with a thick, low doped n-type layer can achieve a lower diffusion current than an analogous structure containing a thick, low doped p-type layer due to the lower diffusivity of minority holes to minority electrons.
Another reason why our novel architecture increases efficiency is that almost all the photocurrent in the thick emitter structure is produced from the emitter, so device performance is very sensitive to the minority carrier lifetime in this layer. It is possible to optimize the emitter doping concentration to ensure high quality material with a close-to-unity internal efficiency, and a long enough diffusion length to provide efficient minority carrier collection.
When the thick emitter is included in a structure with efficient photon recycling "“ such as a device with very high internal efficiency and a high-reflectivity, back-surface reflector "“ this can lead to significant voltage enhancements. In contrast, in a conventional, thin-emitter structure, the dark current and photocurrent contributions of the solar cell are distributed more evenly among the emitter, depletion and base regions of the solar cell, making it more difficult to design a device with both high performance and high sensitivity to photon recycling effects.
Our modeling and experimental efforts have determined the significant increase in voltage output that results from the inclusion of a gold back mirror on our thick emitter GaAs cells (see Figure 5). This device delivers a conversion efficiency of 27.8 percent, within 3 percent (relative) of the current world record for a single-junction solar cell. The performance of this device approaches the fundamental, thermodynamic limit for an ideal GaAs cell.
Figure 5: A variety of models have been used to try and capture the performance of the thick-emitter, single-junction cell that incorporates a reflecting gold mirror at the bottom of the device. The model by Hovel and Woodall fails to accurately reproduce the experimental data. Incorporating multiple reflections from the gold mirror, which is also known as a back surface reflector (BSR) improves the calculation, but both BSR and photon-recycling contributions are required to replicate the experimental results.
We have used a variety of models to predict the performance of this thick-emitter, single-junction cell. Calculations based on the model by Hovel and Woodall underestimate performance, due to a failure to capture the optical enhancement that arises due to the multiple reflections from the back surface reflector, and the suppression of radiative recombination due to photon recycling. Slight improvement results from the inclusion of a more realistic generation function that incorporates multiple reflections from the back mirror and the concomitant increase in the short-circuit current density. However, this model still assumes bulk lifetime values with no photon recycling, giving a diffusion length of 4.3 mm for minority holes in the emitter.
When photon recycling it taken into account, the diffusion length increases to 17.1 mm and the minority carrier lifetime increases. Our model can then capture the increase in photocurrent extraction efficiency and reduction in dark current. Both these improvements impact the light-current-voltage curves, where it is possible to note increases in short-circuit current density and open-circuit voltage. This voltage exceeds that predicted by the Hovel and Woodall model and highlights the need for modeling that includes photon management strategies, which allow solar cells to get even closer to their fundamental performance limit.
-The authors would like to thank Dr. I. Vurgaftman, Dr. A. Hanbicki of the Naval Research Laboratory, J. Adams, V. Elarde, G. Hillier and the R&D team of Microlink Devices Inc., and D. Friedman, I. García and S. Kurtz of NREL for helpful discussions relating to this work. Research at NREL was supported by the U.S. Department of Energy under Contract No. DE-AC36-08GO28308 and funded in part by the Foundational Program to Advance Cell Efficiency.