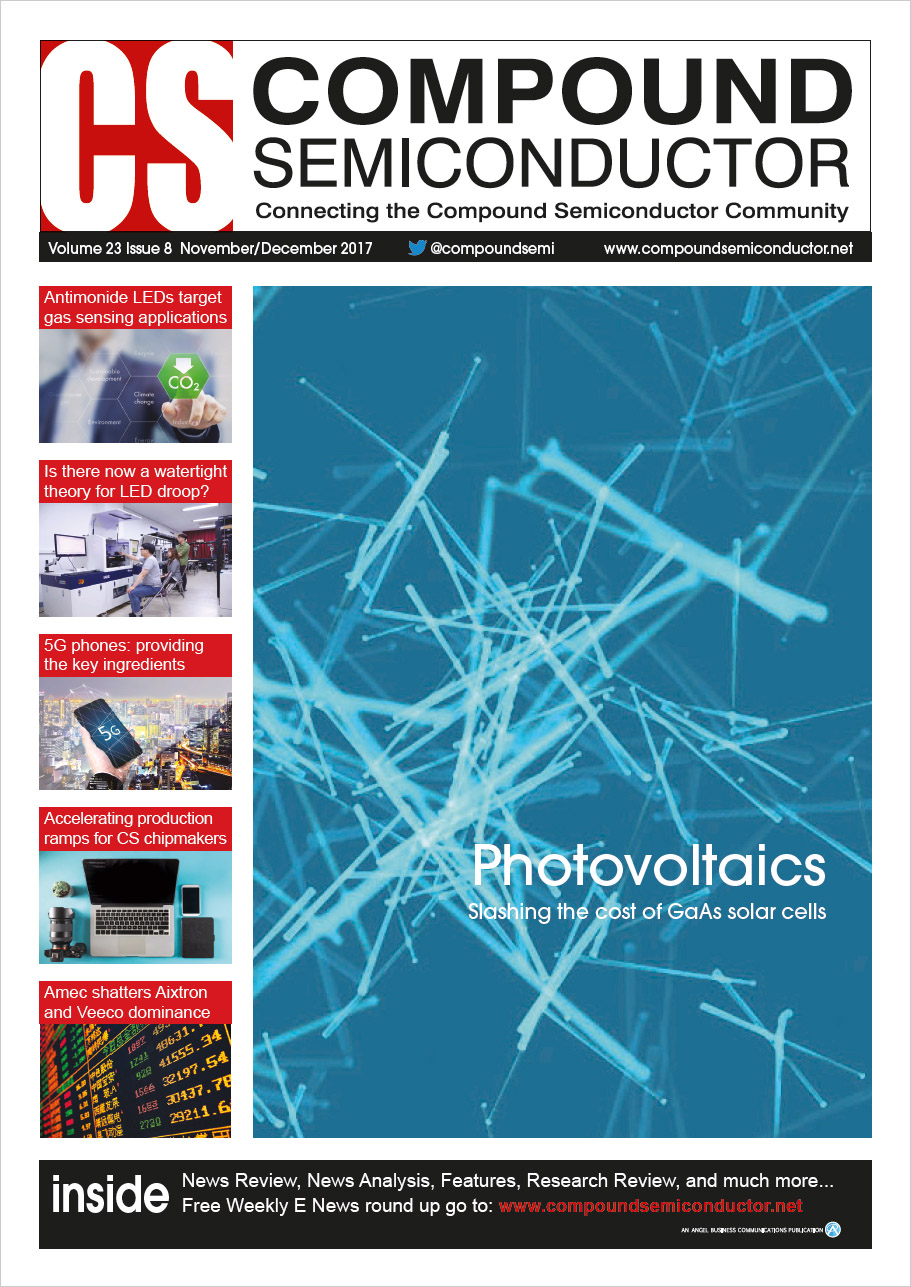
Antimonide LEDs target gas sensing
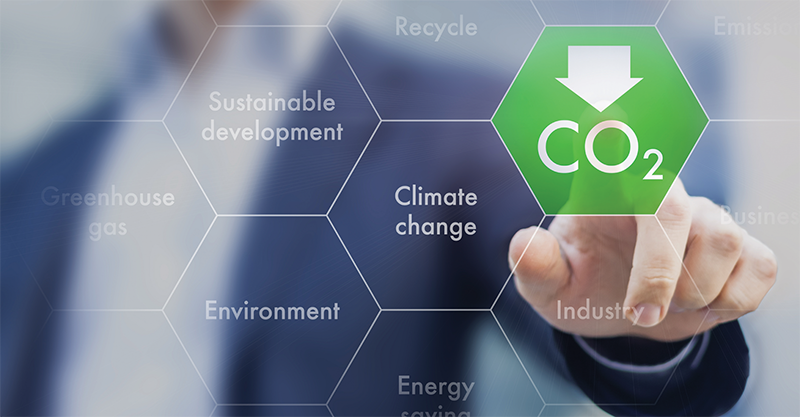
Ventilation systems can consume less power when they incorporate gas sensors with infra-red LEDs delivering ground-breaking performance by Koichiro Ueno, Hiromi Fujita, Osamu Morohara, Edson Camargo, Hirotaka Geka, Yoshihiko Shibata and Naohiro Kuze from Asahi Kasei Microdevices Corporation
To try and stop you from feeling sleepy at work, fresh air is brought into your office through the heating, ventilation and air-conditioning system. However, because this process is not controlled, it often wastes energy. Much better is to introduce just the right amount of fresh air, determined by gauging the quality of the air in the office by its concentration of CO2.
An ideal component for accomplishing this is a CO2 sensor that combines a low power consumption with a high measurement resolution. Both requirements can be meet with a gas sensing technology based on non-dispersive infra-red absorption. Such sensors utilize the absorption of infrared light in the 3 μm to 6 μm spectral range, with specific wavelengths identifying specific gases (see Figure 1). Low power operation may be realised by pairing a photon detector with an LED, operated with a high frequency, low-duty cycle. At Asahi Kasei Microdevices Corporation of Japan we have already developed and commercialised one of these two key ingredients "“ a mid-infrared quantum photon detector. Our device, which is based on an InSb hetero-epitaxial structure, is an attractive alternative to conventional thermal detectors, such as thermopiles and pyro-electric detectors.
Our next step is the development of a high-intensity mid-infrared light source. To this end, we are developing an efficient mid-infrared LED, which is a device that dates back many decades.
One of the key milestones in the development of this device came in the late 1990s, when a partnership between Lancaster University, UK, and the Ioffe Physico-Technical Institute in St. Petersburg, Russia, produced an LED with a strong room-temperature emission at 4.6 μm. This device, suitable for carbon monoxide detection, features an InAsSb/InAsSbP double-heterostructure grown by liquid phase epitaxy. Another key breakthrough from around this era, coming from a team at Grenoble, France, was the development of a resonant cavity structure that improved light extraction.
Figure 1. Infrared transmission spectrum of tested gases. Data source is NIST Chemistry WebBook (http://webbook.nist.gov/chemistry).
More recently, a UK-based team from QinetiQ and the University of Bristol, and another collaboration that has been led by researchers at the University of Glasgow, have both shown that the internal quantum efficiency in this class of device can be increased by turning to strong quantum well carrier confinement structures, such as InSb/AlInSb and GaInSb/AlGaInSb.
Drawing on all of this work, we have developed a high-intensity AlInSb mid-infrared LED. Its key features are InSb/AlInSb double buffer and electron/hole double barrier layers, and a highly efficient, light-extracting backside emission structure.
Figure 2. (a) A top view of the eight series-connected mid-infrared LED chip produce by Asahi Kasei Microdevices Corporation. (b) A cross-section of the AlInSb mid-infrared LED, which is formed by growing a 0.5-μm-thick InSb buffer layer with tin doping at a level of 7 à— 1018 cm-3; followed by a 2.0-μm-thick intrinsic AlxIn1-xSb active layer, sandwiched between the tin doped (n+) and zinc doped (p+) 20 nm-thick Al0.22In0.78Sb hole/electron barrier layers; and finally, a 0.5 μm-thick, Zn doped p+ AlxIn1-xSb top layer (2.0 à— 1018 cm-3). In the top layer, the aluminium concentration (x) is varied from 3.9 percent to 7.6 percent.
We produce our devices by loading semi-insulating GaAs (100) substrates into in Riber MBE 49 tool, thermally cleaning them, and then depositing a heterostructure (see Figure 2 for details). This design provides efficient carrier confinement: there are barrier heights of up to 0.3 eV for holes/electrons, due to the bandgap for the n/p Al0.22In0.78Sb barrier layers being higher than that for the Al0.05In0.95Sb active layer (see Figure 3).
Epiwafers were processed into eight series-connected diodes, using Au/Pt/Ti as the electrodes (see Figure 2(a)). To increase light extraction, the backside of the wafer was thinned and roughened with a conventional back-grinding process, and then covered with a TiO2 anti-reflection coating. Finally, the devices were cut into chips with a 0.5 mm2 area, using a dicing process, and then assembled into a plastic-mould quad flat non-leaded package. It has dimensions of 2.6 à— 1.9 à— 0.4 mm.
Figure 3. Energy band diagram of the Al0.03In0.97Sb mid-infrared LED.
We have recoded the emission spectra for a range of LEDs with different levels of indium content (see Figure 5). As expected, as the aluminium content increases and the bandgap increases, the emission peak shifts to a shorter wavelength.
Compared to a mirror-polished surface, the combination of roughening and deposition of a TiO2 film produces a 25 percent hike in emission intensity (see the inset to Figure 5). Our approach also has the merit of being simple and effective. While other methods can also increase light extraction efficiency, they are difficult to implement with a top-emitting device architecture.
To ensure a high light extraction, we roughen the backside of our device with a conventional back grinding technique. This creates a coarse surface with a root-mean-square roughness of 200 nm. On this roughened surface we deposit a TiO2 insulating film that has two benefits: it forms an anti-reflection coating; and it passivates the GaAs, prevent surface oxidation.
Transmission electron microscopy offers an insight into the quality of our heterostructures (see Figure 4). Due to the large lattice constant mismatch between the InSb buffer layer and GaAs substrate "“ it is 14.6 percent "“ there is the threat that misfits and dislocations at the interface lead to threading dislocations within the film. But that's not the case in our material. Instead, a significant proportion of the threading dislocations are blocked at the AlInSb/InSb buffer layer and at the AlInSb barrier/active layer. Thanks to this, the threading dislocation density in the active layer is limited to 2.9 ± 0.3 à— 108 cm-2. Although that value is quite large compared to a lattice-matched or a pseudomorphic system, it is still quite low for an antimonide-based system heteroepitaxially grown on a GaAs substrate.
Blocking dislocations
Transmission electron microscopy offers an insight into the quality of our heterostructures (see Figure 4). Due to the large lattice constant mismatch between the InSb buffer layer and GaAs substrate "“ it is 14.6 percent "“ there is the threat that misfits and dislocations at the interface lead to threading dislocations within the film. But that's not the case in our material. Instead, a significant proportion of the threading dislocations are blocked at the AlInSb/InSb buffer layer and at the AlInSb barrier/active layer. Thanks to this, the threading dislocation density in the active layer is limited to 2.9 ± 0.3 à— 108 cm-2. Although that value is quite large compared to a lattice-matched or a pseudomorphic system, it is still quite low for an antimonide-based system heteroepitaxially grown on a GaAs substrate.
To ensure a high light extraction, we roughen the backside of our device with a conventional back grinding technique. This creates a coarse surface with a root-mean-square roughness of 200 nm. On this roughened surface we deposit a TiO2 insulating film that has two benefits: it forms an anti-reflection coating; and it passivates the GaAs, prevent surface oxidation.
Compared to a mirror-polished surface, the combination of roughening and deposition of a TiO2 film produces a 25 percent hike in emission intensity (see the inset to Figure 5). Our approach also has the merit of being simple and effective. While other methods can also increase light extraction efficiency, they are difficult to implement with a top-emitting device architecture.
We have recoded the emission spectra for a range of LEDs with different levels of indium content (see Figure 5). As expected, as the aluminium content increases and the bandgap increases, the emission peak shifts to a shorter wavelength.
Figure 4. Cross-sectional transmission electron micrograph of the AlInSb mid-infrared LED structure. This image reveals that threading dislocations do not propagate through the heterostructure.
To benchmark the power produced by our devices, we have compared the power conversion efficiency of our Al0.061In0.939Sb LED with a reference, chosen because it has the highest level of efficiency of all those currently available on the market (see Figures 6 and 7). Our device has the upper hand, delivering about 75 percent higher emission efficiency than the reference.
Figure 5. Room-temperature emission spectra for AlxIn1-xSb LEDs (x=3.9, 5.1, 5.4, 6.1, and 7.6 percent) made by Asahi Kasei Microdevices. Spectral characteristics were measured with a Fourier transform infrared spectrometer with a HgCdTe detector. The device was driven with a peak injection current of 100 mA and a duty cycle set to 5 percent at 2 kHz.
It is not surprising that our LED has a lower power conversion efficiency than its shorter-wavelength cousin, because in the mid-infrared spectral domain, efficiency is known to decrease significantly as wavelength increases. The two primary reasons for this are that Auger recombination is more prevalent in narrow bandgap semiconductors, and that the packaging technology for this class of LED is relatively immature. The packaging technology that is adopted is quite different from that for visual light LEDs, which tend to be housed in bullet-shaped transparent plastic-mould packages with a high light extraction efficiency. Instead, the mid-infrared LEDs are often placed into a metal can package.
Figure 6. Room-temperature emission spectra for an Al0.061In0.939Sb LED made by Asahi Kasei Microdevices and a reference LED.
We have paired our mid-infrared LED with our infrared sensor to create a gas sensor with an optical path length of about 90 mm (see Figure 8). This gas sensor also has an integrated circuit, which has a dual role, driving the LED and providing signal processing from the IR sensor output. With this gas sensor, the calculations for CO2 concentration are based on the extent of the decrease in the infrared sensor signal intensity.
Figure 7. Power conversion efficiency for a range of LEDs, from the ultraviolet to mid-infrared. Data is extracted from commercial LED datasheets. The efficiency for the Asahi Kasei Microdevices' LED, which is estimated from the comparison data with the reference LED in Figure 6, is also shown.
To assess the output provided by our CO2 sensor, we have turned to calibration gases with CO2 concentrations of 0, 1000, 2000, 3000 and 5000 ppm (see Figure 9). During these measurements, LEDs were driven at 100 mA at room temperature, using a duty cycle set to 0.13 percent (the on-time is about 0.2 ms).
Results indicate that the output of our sensor is in good agreement with the calibration gas concentration. We have found that the resolution of our sensor is about 15 ppm at a CO2 concentration of 1000 ppm. Under these conditions power consumption averages below 1 mW, which is, to the best of our knowledge, the lowest power consumption of any currently available non-dispersive infrared gas sensor.
Figure 8. Gas sensor experimental setup. Photographs are for an InSb quantum type mid-IR sensor (AK9710) and a mid-infrared LED assembled in a plastic-mould package (the optical emission window can be observed at the centre of the package, surrounded by four electrode terminals).
Our next step is to develop LED/sensor devices for detecting other gases with absorption wavelengths in the mid-infrared, such as methane and ethanol. That will not only enable our sensors to efficiently provide great air-quality at your desk "“ it will also help detect: leaks of methane gas, which has a greater potential for global warming than CO2; and prevent drunk motorists from driving.
Figure 9. Results of gas sensor output, measured using calibration gases (0, 1000, 2000, 3000, 5000 ppm CO2 concentrations).