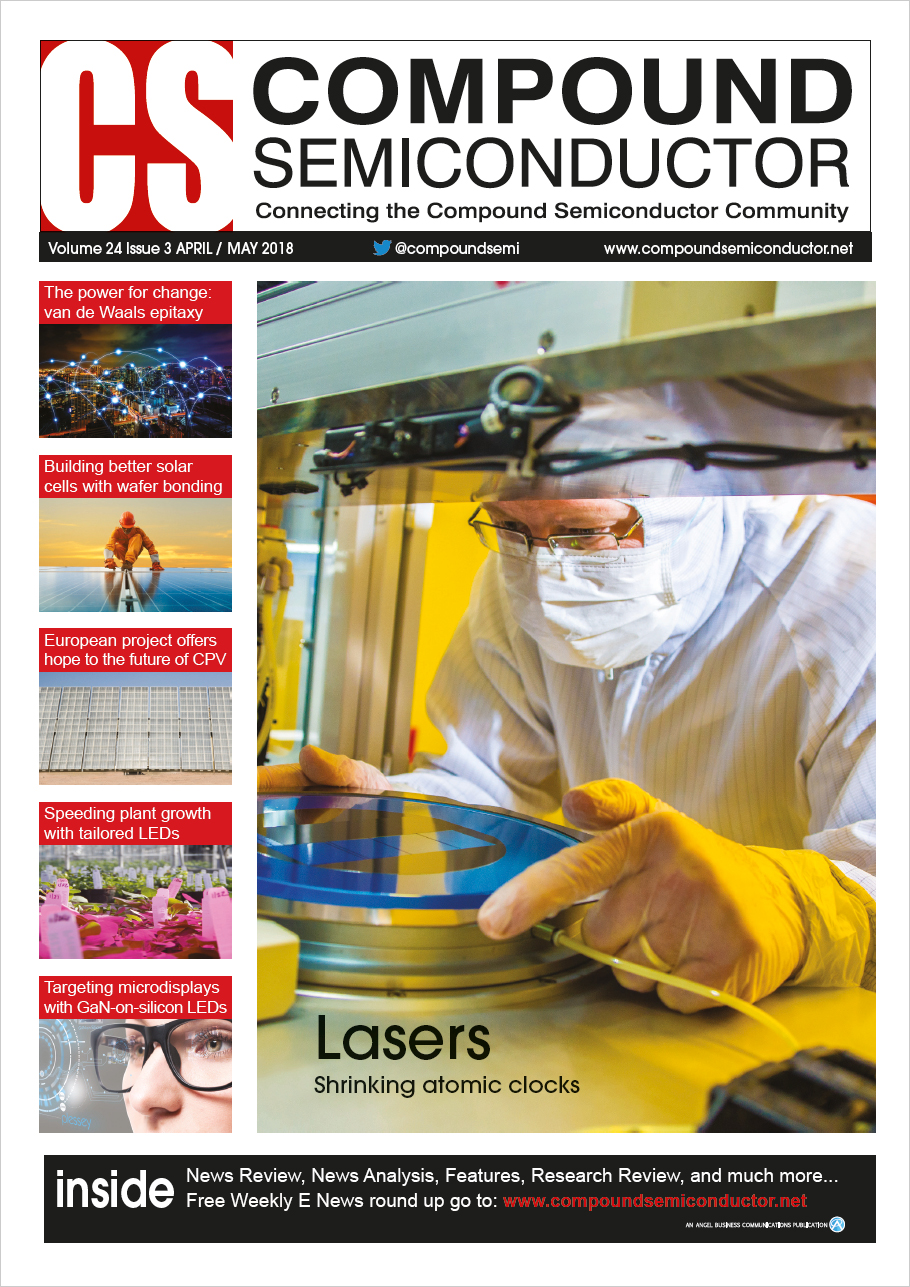
The power for change: van der Waals Epitaxy
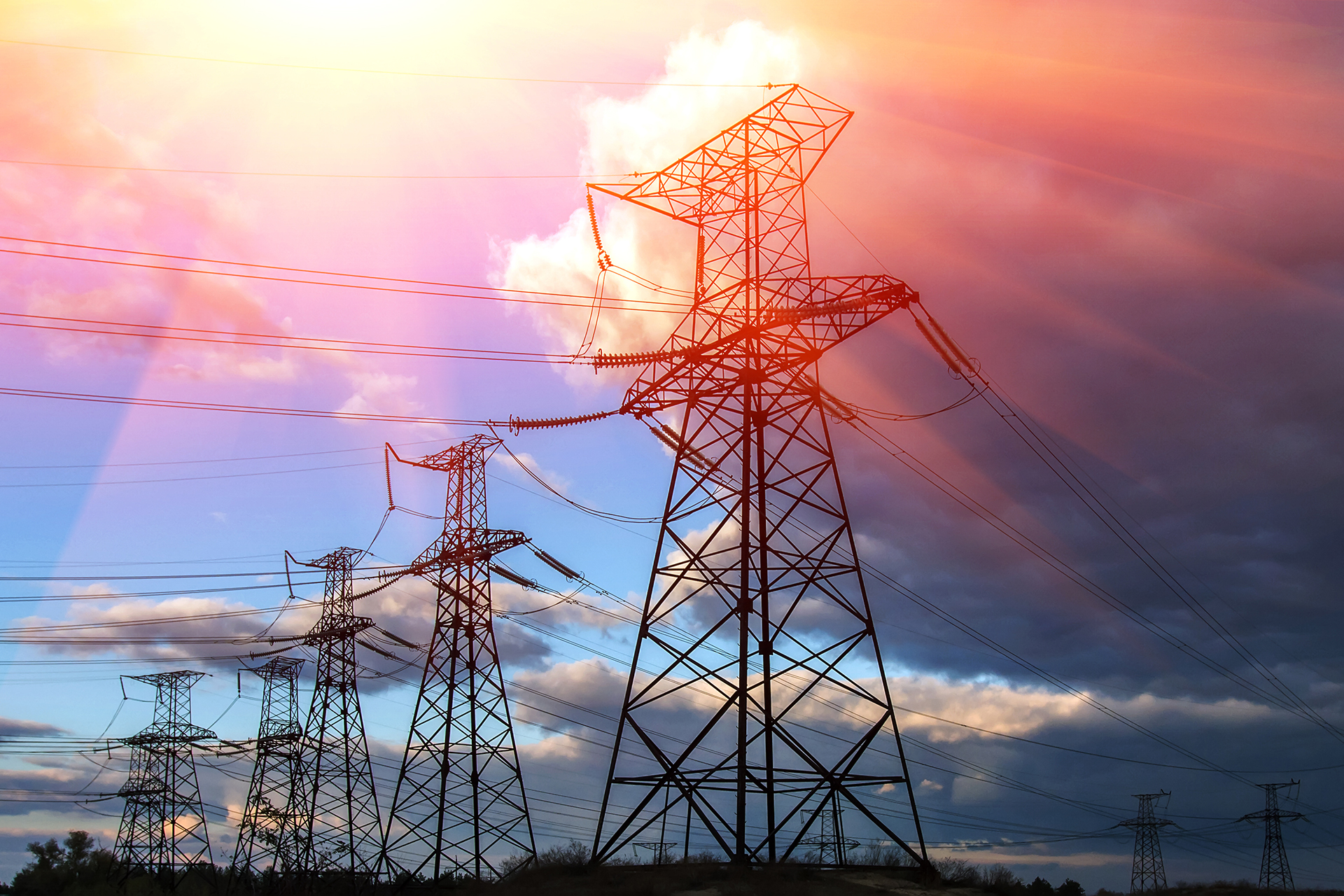
A novel growth process, which forms thin, high-quality layers of GaN on graphene, promises to slash substrate costs and open up new device architectures by can Bayram from the University of Illinois at Urbana-Champaign
As regular readers of this magazine know, III-V compound semiconductors are leaving an indelible imprint on humanity. These materials are used to make LEDs, laser and amplifiers "“ and these devices are lighting our homes, offices and displays; reading our DVDs and Blu-ray disks; and increasing the communication speeds through wireless and wired connections.
The emergence of this multi-billion-dollar industry can be traced back to the 1950s, when extensive studies of GaAs first began. Since then, there has been diversification of materials, with InP research and development taking off in the 1970s, followed by the addition of GaN in the 1990s.
Judged in terms of intrinsic characteristics, GaN, along with its related alloys, is undoubtedly the most promising material system. By tuning the composition of the quaternary InAlGaN, the emission wavelength of the material can span from the deep ultraviolet to the near-infrared; and by subband-energy engineering of AlGaN/GaN superlattice quantum structures, the optical capability of this material can stretch to the terahertz regime. When it comes to electronics, GaN-based compound semiconductors can combine a large bandgap "“ it is 3.4 eV for GaN and 6.2 eV for AlN "“ with a high critical electric field strength, typically 106 V/cm, and high saturated electron drift velocities. Devices are no slouches either: AlGaN/GaN transistors that sport a two-dimensional electron gas are capable of cut-off frequencies in excess of 300 GHz.
However, GaN devices do have an Achilles heel: there is no lattice-matched, low-cost, high-quality substrate. Free-standing GaN can be formed, but not by the crystal growth methods used to make GaAs and InP, and the substrates that result are limited in size and quantity, riddled with imperfections, and very pricey.
Consequently, many devices are grown on foreign substrates that have a symmetry that is similar to that of GaN. Popular choices are sapphire, 4H-SiC, and silicon (111). Deposition of the GaN often occurs by MOCVD, with gallium and nitrogen introduced to the growth chamber in the form of a metal-organic compound and ammonia, respectively. When these materials impinge on the heated substrate, they decompose, and a mix of ionic and covalent forces drive the formation of GaN epilayers.
Figure 1. Bandgap energy versus lattice constant plot for III-V materials. The direct bandgap nature of III-nitrides enables emission tunability from ultraviolet to infrared wavelengths, making them one of the most promising materials for photonics.
While the resulting material is good enough for making commercial devices, it is far from ideal. When GaN is deposited on sapphire, 4H-SiC, and silicon (111), there are lattice-mismatches of 14 percent, 3 percent, and 17 percent, respectively (see Table 1), that lead to threading dislocation densities in excess of 108 cm-2. These imperfections act as non-radiative recombination centres, pegging back the efficiency of LEDs, lasers, transistors, and terahertz oscillators.
Fortunately, there is a low-cost, highly promising solution: van der Waals growth of GaN on graphene, a one atom-thick carbon chain that is cheap, scalable, and abundant. Our team at the University of Illinois at Urbana-Champaign is pioneering this approach, and has succeeded in being the first group to grow single-crystalline single-phase large-area GaN films on graphene by van der Waals epitaxy.
This form of epitaxy is markedly different to that of MOCVD. The starting point is the same "“ ammonia and a metal-organic compound are the reactants "“ but growth relies on the weak atomic forces between the gallium, nitrogen and carbon atoms. Due to this, van der Waals forces dominates the material deposition rather than much stronger ionic or covalent forces.
One of the great strengths of van der Waals epitaxy is that such weak interaction between GaN and carbon drastically relaxes the lattice-matching condition usually encountered in heteroepitaxial growth. The upshot is tremendous freedom in the combination of materials for forming heterostructures. This opens the door to a new way of fabricating novel heterostructures, using ultrathin superconducting, metallic, semiconducting or insulating films of layered materials as constituents.
Figure 2. Researchers at UIUC have pioneered a method for growing/transferring single-crystalline thin films on/from epitaxial graphene. (a) Graphitization of a SiC substrate to form epitaxial graphene. (b) Epitaxial growth of GaN on graphene. (c) Deposition of a stressor layer (Ni). (d) Release of GaN from the substrate with a handling tape. (e) Transfer of the released GaN/Ni/tape stack on a host substrate. (f) Removal of the tape and Ni by thermal release and wet etching, leaving a GaN film on the host substrate. (adapted from adapted from J. Kim et al. Nature Communications 5 4836 (2014) and references there within).
By drastically relaxing the lattice-matching condition, van der Waals epitaxy offers much promise on many fronts. Its potential is not limited to just fulfilling the role of an epitaxial seed and release layer for subsequently-grown layers. If graphene is sandwiched between two other semiconductors, such as SiC and GaN, there is the potential to make hot electron transistors that are suitable for high-frequency, highspeed current switching applications. Calculations by Xiangfeng Duan's group at UCLA suggest that hot electron transistors formed by the combination GaN-graphene-GaN can realise operation at 1 THz. Meanwhile, on the photonic side, when bi-layer graphene is sandwiched between wide bandgap materials, it possesses a bandgap of around 200 meV, an attribute that could aid the production of modulators. And in addition to these more esoteric devices, the pairing of GaN and graphene could form vertical GaN transistors, such as high-speed, highpower HBTs; and LEDs.
Despite this tremendous promise, van der Waals epitaxy of GaN materials on graphene "“ or any other two-dimensional material for that matter "“ has been almost unexplored until recently. That's because much effort has been directed towards conventional materials and their heterointegration.
Encouragingly, dangling bonds were not needed for initial success, with van der Waals epitaxy enabling the direct deposition of a layered material onto a cleaved face of another layered material. And more recently, van der Waals epitaxy has been possible with the deposition of two-dimensional semiconductor structures on both two- and three-dimensional semiconductors, with the process driven by the formation of mixed atomic bonds.
Figure 3. Thin-film blue LEDs via GaN-on-graphene technology. (a) Cross-sectional transmission electron microscopy image of LED stacks (p-GaN/MQW/n-GaN) on a graphene/SiC substrate (scale bar, 1 μm) and high-resolution transmission electron microscopy magnified the multi-quantum well (scale bar, 100 nm). (b) High-resolution X-ray diffraction/simulation data from the InGaN/GaN LED heterostructure grown on a reused graphene/SiC substrate. (c) Photoluminescence data from the InGaN/GaN LED heterostructure grown on a reused graphene/SiC substrate. The picture of the LED-emitting blue light is displayed in an inset. (d) Schematic of a transferred visible LED device on the tape. (e) Current-voltage characteristics of a transferred LED stack measured by applying positive bias on nickel and negative bias on n-GaN. (f) Electroluminescence spectra of a transferred LED stack taken at 10 mA injection. The picture of the LED-emitting blue light is displayed in an inset.
However, when it comes to the growth of threedimensional semiconductors on two-dimensional semiconductors, it's been a different story. These efforts, typically associated with the likes of three-dimensional CdS and CdTe on two-dimensional graphene, MoTe2, and WSe2, tend to suffer from many weaknesses. They include: structural metastability, that is, phase-mixing; chemical metastability, such as a tendency toward spinodal decomposition; and in some instances, including that of GaAs, chemical incompatibility. The root causes are that growth requires elevated temperatures and complex precursors.
Due to these impediments, growth tends to form oriented clusters of polycrystalline material with a grain size of less than 100 nm. These grains are so small that they render this technology useless for the production of substrates and devices. Note that the insertion of an intermediate layer, such as ZnO in-between a compound semiconductor and a twodimensional layered substrate, is not a great solution. That's because it results in low-quality heteroepitaxy, and leads to a partial film coverage at best.
The way forward is to directly deposit a threedimensional semiconductor on a two-dimensional material through a form of van der Waals epitaxy. We have pioneered this using epitaxial graphene as the platform. Epitaxial graphene is enabled through sublimation of a SiC substrate at high temperatures, typically more than 1600 °C). Silicon diffuses out, and the remaining carbon atoms rearrange to enable a self-terminating monolayer graphene, called epitaxial graphene.
Regrowth of GaN on such graphene requires a new epitaxial approach. Instead of inserting a conventional buffer layer at a low temperature of typically 500-700 °C, similar to what have been done conventionally between highly mismatched materials, we employ a high temperature buffer layer at around 1100 °C. Raising the temperature ensures better coverage of the graphene nucleation surface with the buffer layer "“ and when the subsequent growth of GaN takes place, initiated from the graphene facets, heteroepitaxy evolves properly.
One of the strengths of this GaN on epitaxial graphene approach is that the weak, quasi van der Waals forces between GaN and graphene offer a convenient means for substrate-scale release. This could aid efforts associated with the fabrication of thin, large-area vertical GaN devices as well as reuse of the expensive SiC substrates.
Our technology has led to the growth, on epitaxial graphene, of GaN films with a root-mean-square roughness of 3 à… and a defectivity as low as 4 à— 108 cm-2. We have also been able to transfer entire single-crystalline GaN films on to an arbitrary substrate (see Figure 2).
What's more, because graphene is flat, so is the released interface "“ it has a root mean square roughness of just 5 à…. Thanks to this, we have been able to form adhesive-free, GaN-on-silicon structures that are ideal for the production of hybrid GaN-onsilicon (100) devices. By re-using the graphene/SiC substrate, we have been able to undertake multiple growth and transfer cycles of GaN films.
One of the key milestones in our work has been the growth of InGaN/GaN epitaxial LED stacks on a recycled graphene/SiC substrate "“ it has been reused three times. The fully-functional flexible blue LEDs that we have demonstrated (see Figure 3) are ultra-light and are capable of conforming to curved surfaces.
A major implication of our work is that it has the potential to address one of the biggest challenges facing many forms of GaN power devices, including RF transistors: heat extraction. Our ultra-thin GaN technology allows us to optimize the passive cooling of GaN HEMTs and reduce the junction temperature by around 50°C for 8 W of dissipated heat. Given the exponential relationship between the junction temperature and lifetime, such passive cooling through thickness optimization creates an opportunity for improved output power and reliability.
Table 1. Comparison of crystalline quality of GaN grown on various substrates (adapted from J. Kim et al. Nature Communications 5 4836 (2014) and references there within).
Our results pave a promising path for the development of III-nitride semiconductors, by enabling the integration of these devices with other semiconductors. Further progress hinges on realising a better understanding and greater control of GaN epitaxy on graphene, so that it is possible to produce single-crystalline, wafer-scale GaN material with low defectivity on a scalable graphene platform.
Where we have led, many others will follow, each taking a different path. There are more than five hundred two-dimensional materials, and it is safe to predict that this century will depend as much on twodimensional materials as the last century depended on three-dimensional ones.