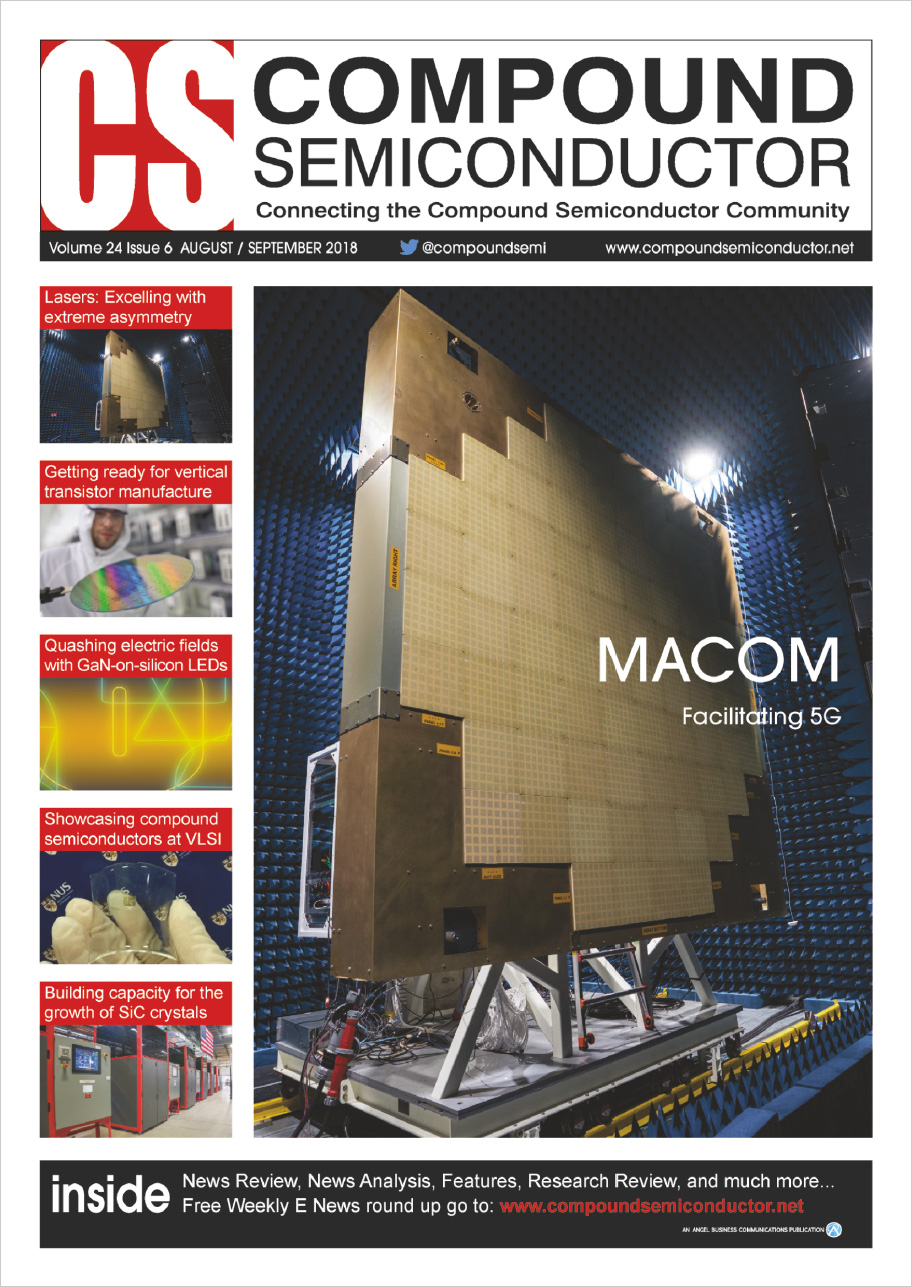
Lasers: Excelling with extreme asymmetry
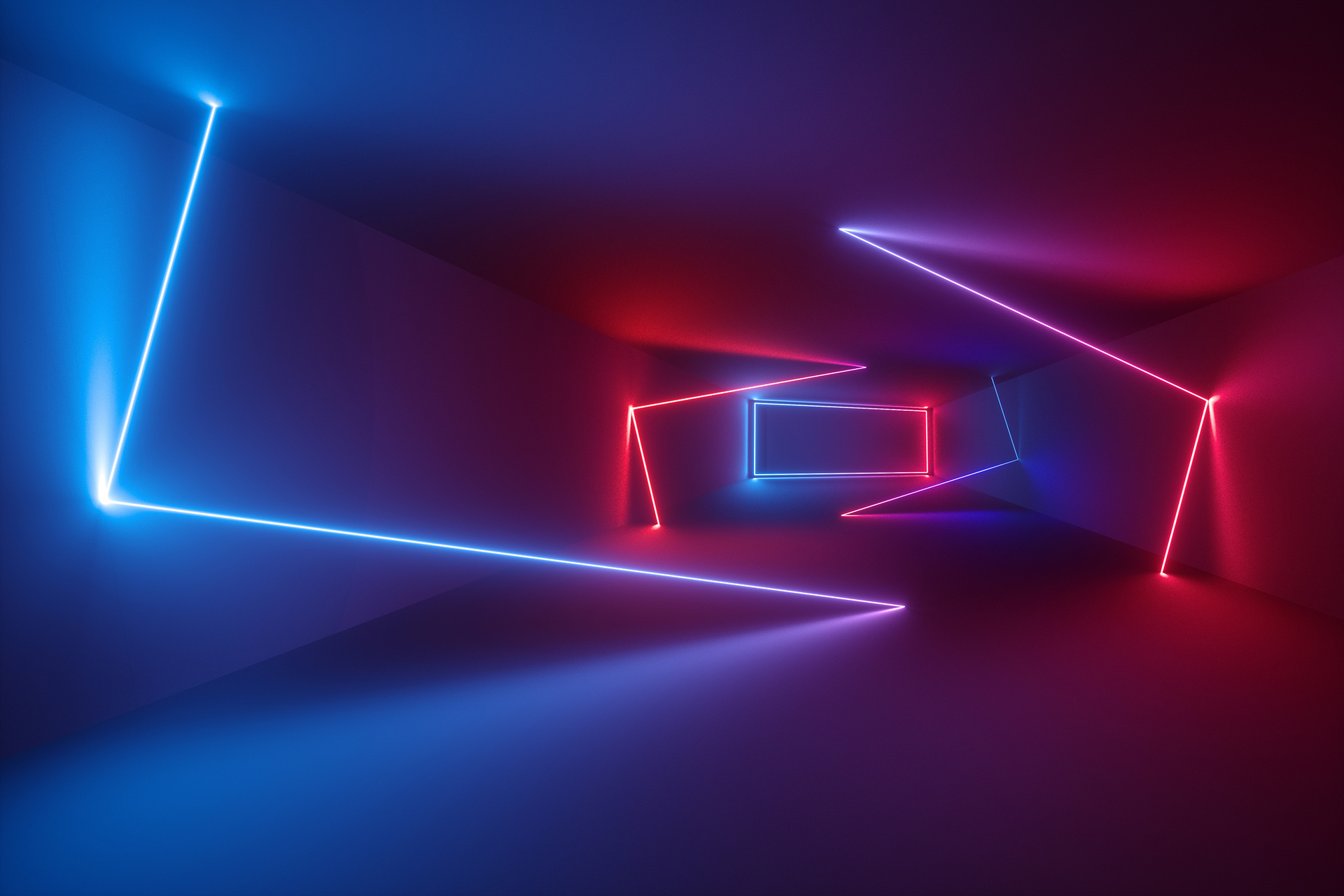
In advanced industrial laser systems, which have uses that include the cutting of metals, highpower diode lasers are the source of all optical energy. In these systems, a highly intense beam is formed by either combining the optical output of many diode lasers, or by using many of them to pump a solid state or fibre laser.
Diode lasers are widely used for this task, because they are the most efficient technology for converting electrical input energy into application-ready light. However, given their key role in industrial laser systems, even higher efficiencies are preferred, along with an increase in the maximum output power. Succeeding on both these fronts requires a good understanding of the physical effects that limit performance, because this can help to guide the development of ever more sophisticated and higher performing device designs. Such efforts should focus on high-power diode lasers that are grown on GaAs substrates and emit in the 900 nm to 980 nm wavelength range, because these are the highest performing devices, and the ones in greatest demand from industry.
At the FBH Berlin, our team has devoted many years to realising a greater understanding of this class of laser, and increasing its performance. GaAs diode lasers are a very mature, highly commercially successful technology. In spite of this, there is still much to learn and substantial improvements are still possible.
Recently, we introduced sophisticated epitaxial layer structures that have increased the power and efficiency of this infra-red source. These groundbreaking devices employ extreme triple asymmetrical designs that precisely manipulate the optical field within the chip.
Figure 1. Left: Modern epitaxial growth tools enable sophisticated extreme triple epitaxial structures to be precisely and reproducibly grown on GaAs wafers, as needed to deliver ever higher powers and efficiencies. (Image © Maria Vittoria Trovato). Top right: Epitaxy wafers are fabricated into high-power laser bars, with geometry tailored to the application, shown here ready to be packaged for pumping or direct applications (Image © FBH/Schurian.com). Bottom right: Highpower laser bars mounted in a passively cooled heatsink, suitable for use in kilowatt-class pumping applications, where the highest power and efficiency are needed (Image © FBH/Schurian.com).
Our design is a modification of a conventional highpower GaAs diode laser (see Figure 1), which is typically grown by MOCVD. Dimensions of a standard large-area chip are a 4 mm and 6 mm length and an emission aperture of about 90 μm to 100 μm. Fabrication uses advanced III-V process techniques, such as facet passivation, while overheating is prevented by soldering the chips to a well-cooled highly conductive heatsink. High optical output is not necessarily an impediment to high reliability, and the output of the best chips is limited by fundamental loss mechanisms, rather than process or growth-related defects.
Figure 2. Conventional high-power diode laser
In all diode lasers, applying an external bias voltage drives electrons and holes towards a quantum well that is 5 "“ 10 nm thick. Here they are captured, and recombine to generate and amplify light. In conventional laser designs the well is surrounded by a thick, low-doped waveguide layer, itself sandwiched between two cladding layers with a lower refractive index. This architecture ensures that the optical field is confined in a region close to the quantum well (see Figures 2 and 3).
Figure 3. A conventional diode laser design has many inherent limits. (a) Vertical refractive index profile and optical field profile. More than 90 percent of the electrical resistance is from the p-side. When driven at high bias (here, 40 A in a laser with a 200 μm stripe and a 4 mm resonator), the p-side waveguide deforms, and many electrons escape and accumulate, dramatically increasing losses. This is seen in (b) the simulated vertical band structure and (c) carrier density. For more details see K.-H. Hasler et al. Semicond. Sci. Technol. 32 045004 (2017)
One of the weaknesses of these conventional highpower diode laser structures is the large difference in properties of n-type and p-type material, which supply the electrons and the holes, respectively. Specifically, the p-type material has a resistance that is ten to twenty times higher than the n-type, and an absorption that is four times higher. That is, the materials are highly asymmetric in their properties, but the vertical designs are not. Due to these imbalances, even well-optimized symmetric layer designs suffer from either high optical losses or high electrical resistance. Making matters worse, when devices are driven at high currents, a large voltage is needed to flow current through the resistive p-side waveguide. This directly limits the peak achievable power: the high voltage drop leads to band-bending, and this allows many electrons to escape from the well. When they exit, they accumulate in the p-waveguide, where they are rapidly lost. This contributes to optical losses, and limits the power produced by the chip.
Asymmetry, asymmetry...
As a first step to addressing these losses, we introduced the extreme-double-asymmetric epitaxial layer design (see Figure 4). It features two strong vertical asymmetries. The first is a thin p-waveguide "“ making it as thin as possible slashes the optical loss and electrical resistance. The second is a very different composition for the cladding layers either side of the waveguide, a modification that couples unwanted optical modes into the substrate. An additional merit of this design is that the thin p-waveguide prevents electron escape at high bias. Armed with this architecture, lasers have lower loss and resistance, and produce higher output powers (see Figure 4).
Figure 4. Extreme-double asymmetric (EDAS) structures improve performance, but problems remain. (a) Vertical refractive index profile and optical field profile. Electrical resistance from the (very thin) p-side is strongly reduced. (b) Electrical resistance is low, power saturation is improved, shown here for EDAS and conventional devices lasing close to 940 nm, with narrow stripes (32 μm) and long resonators (6 mm).
However, although performance was improved, our patented design needed further development. This double-asymmetric approach produces an offset between the optical field and the quantum well, lowering gain and leading to an increase in threshold current and a lowering of peak conversion efficiency. In addition, in spite of suppressing key power saturation effects by design, diode lasers using double asymmetry deliver significantly lower powers than predicted, even when studied using the best calibrated and most detailed simulation tools.
...and more asymmetry
To address all these weaknesses, we have recently introduced a third degree of asymmetry: an asymmetric refractive index profile for the layers either side of the quantum wells (see Figure 5). Thanks to this modification, the profile of the optical field can be finely tailored. Shifting the optical field towards the quantum well increases optical gain without the need to adjust the p-side, which can remain thin. The upshot is that, for the first time, it is possible to simultaneously realise low resistance, low loss, low power saturation and a low threshold current (see Figure 6).
Figure 5. Extreme-tripleasymmetric structures (ETAS) further improve performance. (a) Vertical refractive index profile and optical field profile of a reference EDAS design. Gain is low, as the optical field has only small overlap with the quantum well. (b) Vertical refractive index profile and optical field profile of a new ETAS design: further asymmetry around the quantum well increases gain, without need to compromise elsewhere.
The complexity of our design, and the need for many finely tuned variations in material composition, does not imply that our laser is destined to remain in the lab. Using modern MOCVD tools and best scientific and engineering practise, fabs can reliably and reproducibly produce lasers with our design. It helps that they are constructed with the long-established GaAs-AlxGa1-xAs material system.
Our flexible mode-shaping technology, for which we have applied for a patent, is capable of producing further performance benefits (illustrated in Figure 6). Fine-tuning the vertical profile of the extreme-tripleasymmetric epitaxial structure allows the amount of light in the quantum well to be selected. It can be quantified using the optical confinement factor. When this increases, the threshold current falls and modal gain increases, leading to much lower temperature sensitivity. This attribute is highly valued in applications such as metal cutting, where the laser must operate at high temperatures in CW mode. The higher optical confinement factor obtained in devices using an extreme-triple-asymmetric epitaxial design also allows these devices to deliver higher optical powers in pulsed mode, as needed for applications like LiDAR. We are currently using both of these benefits of a higher optical confinement factor to realise higher-performance diode lasers. One of our findings may raise a few eyebrows, given that diode laser designs with high optical confinement factors are typically expected to show lower optical powers in pulsed mode, not higher.
Figure 6. Experimentally demonstrated performance benefit of extreme-triple-asymmetric structure (ETAS) lasers. (a) Optical power, voltage and efficiency tested CW at 25°C for chips lasing at 940 nm with a 4 mm resonator and a stripe width around 90 μm. ETAS designs with an optical confinement factor of around 0.5 percent have the same threshold and power as conventional lasers and the same voltage as EDAS lasers, increasing peak efficiency (here to 69 percent) and efficiency at high power (here to 66 percent at 10 W). Prototype ETAS lasers with higher optical-confinement factors also show higher performance, with (b) lower temperature sensitivity (threshold and slope vary more slowly by a factor of about two) and (c) higher pulsed output powers.
The expectation is that high confinement leads to extra absorption at high bias, due to non-linear processes such as gain compression and twophoton-absorption. But our experiments show that for our designs, a high optical confinement factor and a low threshold current enable an increase in power. Or, to put it another way, the benefit of low threshold current "“ and hence a lower carrier density in the quantum well "“ more than compensates for any additional non-linear losses.
It is clear that our advanced extreme-triple-asymmetric epitaxial layer structures can set a new benchmark for the optical power and conversion efficiency of highpower diode lasers. Our technology allows the optical field to be finely tuned, enabling a low threshold to be combined with a low resistance, low loss and low power saturation. Our design is still at an early stage, and we expect that refinements to the laser and a better understanding of the power saturation mechanisms will spur performance to a new level.