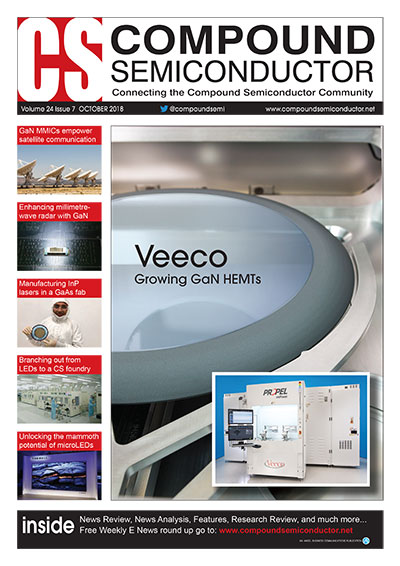
GaN empowers satellite communication
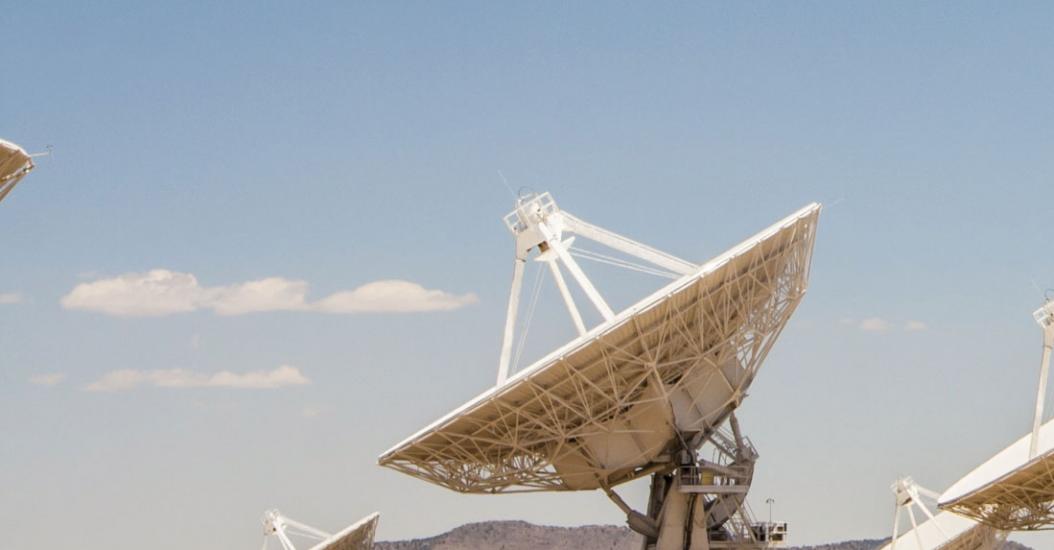
Throughout the history of radio communications engineers have craved more transmit power. This statement is as true today as it was in Marconi's lifetime.
One sector where there is a particularly strong desire for higher transmit power is satellite communications. Here high-power amplifiers and block upconverters have to generate signals that are powerful enough to offset path loss and spreading. In a traditional satellite communication link (see Figure 1), microwave-frequency radio signals are transmitted from a point on earth to a distant satellite in a geostationary orbit that is 35,000 km away "“ that's a distance of almost six times the earth's radius.
Figure 1. Uplink transmitters for satellite communications perform two main functions: frequency upconversion and amplification. This package is often called a block upconverter (BUC). It accepts a low-power, low-frequency modulated signal from a satellite communication MODEM, and upconverts the signal to the specified uplink frequency band; in this example the output frequency is Ku-band, but X-band and Ka-band BUCs are popular as well. A power amplifier (PA) boosts the upconverted microwave signal to the output needed for the satellite communication link. Here the transmitter power is 60 W, but higher power terminals are possible. Thermal management is important to ensure that the electronics do not overheat. The prime power to the block upconverter could be an AC or DC voltage, depending on the application. Monitor and control signals provide telemetry between the block upconverter and the user. Finally, the entire system must be enclosed in a rugged, weatherproof package.
The most common frequency domains for the satellite communications uplink are X-band (7.9-8.4 GHz), Ku-band (13.75-14.5 GHz), and Ka-band (29-31 GHz). Transmitter output power at these frequencies needs to be high, because satellites receive only a miniscule fraction of the power transmitted in their direction. Typically, a communications satellite may receive just a few picowatts of power from an earthbound transmitter that broadcasts tens of watts.
A weak signal reaching the satellite's receiver is not the only issue, however. That signal is coming from a single spot on the surface of the earth that could be masked by the background noise from our warm blue planet, alongside plenty of other potentially inferring signals.
Improvements to the link can result from increasing the power of the transmitter, as this allows the satellite's receiver to "˜hear' signals that appear much "˜louder'. But that's not all. High powers also equip the engineer with greater flexibility when they design the link. Armed with a higher transmit power, engineers can create links with higher data rates, by employing larger bandwidth carriers with more complex modulation schemes to encode the data onto the microwave carrier. In addition, higher transmit powers increase the ruggedness of the link "“ even when it is raining, attenuating the microwave signal, the link is still available. And last, but by no means least, increasing the output power of the transmitter allows a shrinking of the aperture size of the uplink antenna, and ultimately a more compact, mobile platform.
The latter strength is a big deal in today's satellite industry. That's because many satellite terminals are no longer fixed to the ground. Instead they are mobile, serving airborne, maritime, vehicular, and tactical military applications.
This mobility is essential for several new satellite communication networks that are under development, and employ constellations of low- or medium-orbit satellites, which need to be tracked as they move across the sky.
For satellite communication platforms that have to be mobile, or rapidly moving, key attributes for the transmitter are that it is small, lightweight, and draws minimal power. Success on all these fronts ensures that the transmitter is easy to move, and that the link is provided with great power efficiency.
The demand for a higher transmit power is often at odds with the requirements for mobile applications. Generally, higher-power transmitters tend to be larger and heavier, and often less efficient. That means that they tend to need more power to operate, and dissipate more heat.
Fortunately, a step-change in performance "“ in terms of size, weight, and output power "“ is possible by replacing the incumbent technology with new designs based on GaN. Switching from GaAs, the outgoing solution, to GaN is enabling solid-state power amplifiers to combine high efficiencies with impressive output powers while maintaining very compact form factors. And as GaN technology matures, this trend is sure to continue.
Figure 2. Typical layout for a single GaN-on-SiC transistor. There are eight gate fingers with a width of 75 µm,creating a 600 µm device. Several devices are power-combined in the final output stages of GaN MMIC power amplifiers.
Even higher powers can come from uniting several transistors with on-chip combiners (see Figure 3 for an example of an eight-way combiner). Adopt this approach and a single-chip GaN MMIC can produce an output power of more than 60 W in X-band and 20 W in Ka-band.
Thanks to the high power density of GaN, even modest-sized transistors can provide substantial output. For example, a single device with eight gate fingers and 75 mm of gate width can generate over 3 W of output power (see Figure 2).
Many chipmakers are manufacturing such devices. At Qorvo, Wolfspeed/Cree, Northrop Grumman Aerospace Systems, HRL Laboratories, BAE, and WIN Semiconductor, device fabrication is routine, with published amplifier data revealing that power levels are in the 3.0 W/mm to 5.0 W/mm range. At lower frequencies, even higher power densities are possible, with values exceeding 10 W/mm at supply voltages of 50 V or more.
Realisation of higher output powers has hinged on several breakthroughs: T- and G-gate technology; the introduction of the field plates, which holds the key to higher-breakdown voltages; and the global push for higher data rates and more output power from the terminals.
For X-, Ku- and Ka-band frequency satellite communications, GaN HEMTs, grown on SiC substrates, typically have gate lengths of 150 nm to 250 nm. Shrink this and higher frequencies are possible. Reducing to a 90 nm gate length enables operation in the millimetre-wave domain (greater than 30 GHz), and even higher frequencies have been reported for gate lengths as short as 20 nm.
The key device for GaN is the HEMT. It is the building block for the production of GaN MMICs, which provide more output power per die than entire block upconverters built using older, last-generation GaAs technology and designs.
GaN MMICs: Providing the power
The key device for GaN is the HEMT. It is the building block for the production of GaN MMICs, which provide more output power per die than entire block upconverters built using older, last-generation GaAs technology and designs.
For X-, Ku- and Ka-band frequency satellite communications, GaN HEMTs, grown on SiC substrates, typically have gate lengths of 150 nm to 250 nm. Shrink this and higher frequencies are possible. Reducing to a 90 nm gate length enables operation in the millimetre-wave domain (greater than 30 GHz), and even higher frequencies have been reported for gate lengths as short as 20 nm.
Realisation of higher output powers has hinged on several breakthroughs: T- and G-gate technology; the introduction of the field plates, which holds the key to higher-breakdown voltages; and the global push for higher data rates and more output power from the terminals.
Many chipmakers are manufacturing such devices. At Qorvo, Wolfspeed/Cree, Northrop Grumman Aerospace Systems, HRL Laboratories, BAE, and WIN Semiconductor, device fabrication is routine, with published amplifier data revealing that power levels are in the 3.0 W/mm to 5.0 W/mm range. At lower frequencies, even higher power densities are possible, with values exceeding 10 W/mm at supply voltages of 50 V or more.
Thanks to the high power density of GaN, even modest-sized transistors can provide substantial output. For example, a single device with eight gate fingers and 75 mm of gate width can generate over 3 W of output power (see Figure 2).
Even higher powers can come from uniting several transistors with on-chip combiners (see Figure 3 for an example of an eight-way combiner). Adopt this approach and a single-chip GaN MMIC can produce an output power of more than 60 W in X-band and 20 W in Ka-band.
Figure 3. An example of a final stage of a GaN MMIC power amplifier eight-way combiner. Eight transistors (left) are power combined, supplied with DC and routed to the single output (right).
The availability of these incredibly powerful GaN MMICs enables the construction of power-combined block upconverters delivering output powers that were previously unimaginable. What's more, these powerful systems are made from fewer parts than their GaAs cousins, and deliver greater efficiency. Thanks to these strengths, there is no doubt that GaN MMIC power amplifiers will be the main driver in solid-state satellite communication block upconverters for many years to come.
Great power in a small package
At Mission Microwave of Santa Fe Springs, CA, we have built a line of block upconverters for satellite communications around these powerful, efficient
GaN MMICs. A prime example of this is our 100 W, Ka-band block upconverter that is 14 cm in diameter, 34 cm in length and weighs slightly over 4.5 kg (see Figure 4). In comparison, state-of-the-art GaAs Ka-band block-up converters from five years ago could only generate half that power "“ and they did so from a unit that's three times as large and heavy. What's more, our 100 W converter draws less than half of the power from the prime supply.
Figure 4. Mission Microwave's satellite communication block upconverter portfolio. From left to right, 200 W, 100 W, and 25 W Ka-band block upconverters that can efficiently provide linear output powers in industry-leading compact packages. At Ku-band, similarly-sized upconverters can provide 200 W, 100 W, and 55 W of output power.
The differences can be even more striking in the Ku-band. Operating in this spectral range is our 25 W "˜Flatpack' amplifier, designed to be thin enough to be inserted directly into a modern flat satellite communication terminal for applications requiring either high mobility or the ability to track a moving satellite (see Figure 5). Competing block upconverters are up to four times larger than this unit. By trimming size and weight, while upping efficiency, our block upconverter is enabling a new generation of compact, mobile satellite communication terminals for both commercial and military applications.
The key to building these compact, powerful block upconverters is to use a highly integrated design. As output power requirements tend to exceed those produced by a single MMIC, we use a hierarchy of power-combining manifolds with low losses. This preserves efficiency.
Today's GaN MMICs are more efficient than their predecessors, but due to operation at far higher power densities, effective thermal management is critical to ensuring that chips maintain reliable operation. To accomplish this, we undertake thermal, electrical and mechanical design together, in order to optimize the form factor. Other steps that we take to maximise performance are: design the upconverter and signal processing circuitry, to ensure that these components are integrated properly into the unit; custom design the power supply, monitor and control circuitry around the GaN-based power amplifier; and design custom circuitry to extend the useful operating power of our block upconverters.
Figure 5. Mission Microwave's block upconverter. This 0.8 kg, 25 mm-thick package can be integrated directly into a flat-panel antenna system.
Leading with linearity
Designers of power amps love to quote saturated output power because this is the biggest number. But it is not the most meaningful one in a radio communication system, because it is the fidelity, or linearity, of the amplifier that limits usable power. Ideally, the amplitude and phase of the output signal of the power amp are independent of the power level. In practice, however, amplifiers saturate, preventing the output from exceeding a hard-limited maximum, or "˜saturated', power. Complicating matters, the amplitude and phase of the amplifier's gain changes with respect to the signal level, especially near saturation. The downside is nonlinear distortion of the output signal, leading to of out-of-channel interference and increased bit error rates.
These drawbacks are a big deal. Transmitters are moving to higher and higher data rates, enabled by operators turning to ever more complex modulation schemes. With these data transmission technologies, linearity is at a premium. To meet requirements, block upconverters are "˜backed off' from their maximum output power. This move meets linearity requirements and avoids creating signal impairments, which can either degrade link performance or potentially interfere with signals using adjacent channels.
Unfortunately, however, GaN-based amplifiers can be especially susceptible to nonlinear distortion; despite having high saturated output powers, linear output powers of early GaN block-up converters were no better than those of their GaAs-based counterparts. But there is some good news: the linearity of the amplifier can be improved with linearizer circuitry. Many techniques are available, all striving to reduce the dependence of the amplifier's gain magnitude and phase on the drive level.
A common approach for characterising the linear output power of an amplifier that is used for satellite communication is to consider the third-order intermodulation distortion curves. They are produced by feeding in to the unit two equal-amplitude tones, separated in frequency by a few megahertz. Any nonlinear distortion is exposed as additional tones appearing in the output spectrum. Typically, for satellite communication distorting tones should be at least 25 dB below signal tones.
Using this approach to evaluating linearity, an ideal amplifier must be backed off from saturation by just over 2 dB (see Figure 6). In comparison, an unlinearized amplifier, exhibiting a rather "˜soft' gain saturation that is typical of a GaN-based amplifier, has to be backed off by over 9 dB to meet the desired linearity. Far better, though, is our linearized amplifier. This is within 1 dB of ideal, increasing the useful output power by 6 dB over the unlinearized variant.
Figure 6. GaN-based amplifiers require linearization to extend their useful output power.
Pushing to higher powers
We are not resting on our laurels, but continually striving to fulfil the demand for ever increasing transmitter output powers. Recently, we have built a block upconverter that delivers 400 W of saturated power in the Ku-band (see Figure 7).
To evaluate the linearity of this amplifier, we have used another common metric for satellite communication amplifiers: spectral regrowth. For this test, the block upconverter transmits a digitally modulated waveform, just as it would in a real application. Chosen is Offset Quadrature Phase Shift Keyed (OQPSK) modulation, at 10 million symbols per second.
If there is no distortion in this test, the output spectrum of the amplifier will appear as a flat rectangular pulse, with very little power at frequencies offset by more than one symbol rate from the centre. Increase the output power, though, and distortion will kick in. Power will begin to grow outside this main channel, and the spectrum will appear to grow shoulders.
For a typical specification for a satellite communication amplifier, the block upconverter will produce spectral regrowth of less than 30 dB below the main channel at a frequency offset of one symbol rate from the centre. For our 400 W amplifier, this threshold is crossed at an output power of approximately 54.5 dBm (280 W) "“ this is backed off by less than 2 dB from the 400 W saturated output. Note that we realise similar results with our 200 W units operating in the Ka-band. Our next steps are the designing and testing of units that are capable of delivering 800 W in the Ku-band and 400 W in the Ka-band. Were it not for GaN-based MMICs, these power levels would be impractical in a commercial solid-state transmitter.
This will not be the limit of what is possible, as engineers are continuing to make advances in semiconductor devices and materials, propelling operating frequencies and breakdown voltages to new highs. There are also efforts underway to deal with the ever-increasing heat dissipation, including growing the GaN layers on high-thermal-conductivity substrates like diamond, or integrating microfluidic cooling channels into the chip. Such efforts will result in more and more output power from GaN-based MMICs, which will in turn enable higher-power communications transmitters.
Our team can be guaranteed to put this power to good use. Demands for connectivity are only going to increase, along with requests for smaller mobile terminals, which could open the door to sales in new sectors, such as maritime, aviation, consumer, commercial, and government markets.
Figure 7. Mission Microwave's 400-W Ku-band block-up converter generates unrivaled output powers in an industry-leading form factor.