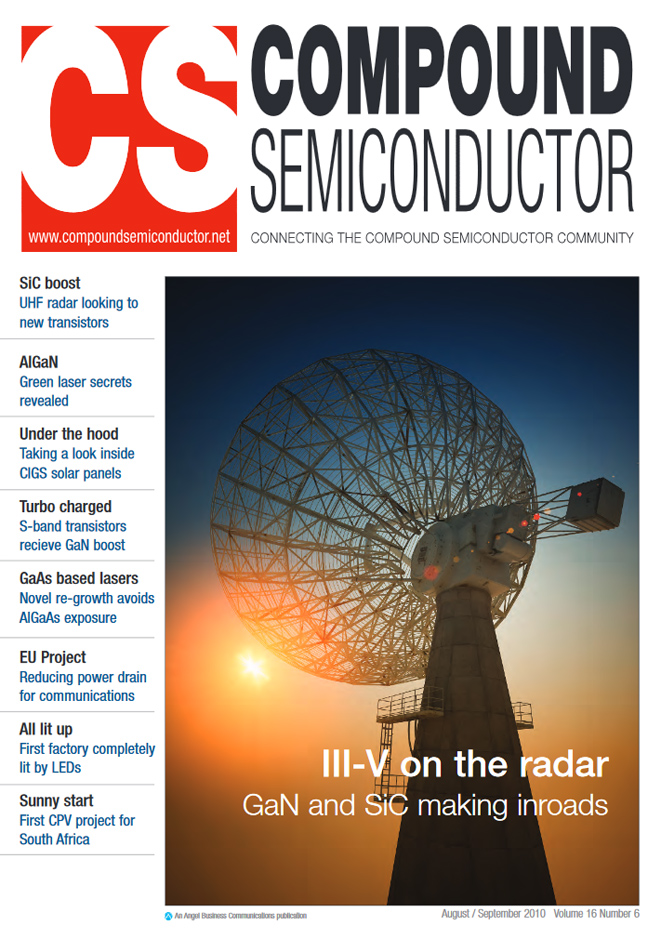
GaN HEMTs advance to ultrahigh bandwidths
A fundamental trade-off between power handling and bandwidth underpins all semiconductor devices: smaller devices are faster, but they handle lower voltages and currents. In short, power roughly scales with the inverse of the frequency squared.
We as device physicists have some flexibility in the face of this trade-off, to the extent that we can play with the composition of the materials to improve breakdown properties. However, there is no free lunch! Typically, materials that can withstand large electric fields show slower moving electrons, precisely because electrons must be tightly held in these materials for them to not breakdown in high electric fields. As someone famous once said, this follows from the "Law of Conservation of Trouble," and it explains why some Compound Semiconductor readers get paid the proverbial "Big Bucks" to do what they do!
Within the array of III-V compounds, GaN stands out because it can deliver bandwidths matching or exceeding those of GaAs devices, with at least five times the output power density per unit device width (1-2 W/mm in GaAs to at least 5-10 W/mm in GaN).
Power at high frequencies is at a premium, and people will buy it, if someone can generate it. There are many angles from which to justify the extension of GaN HEMTs to higher frequencies, and all have to do with the power/frequency trade-off. As a rule of thumb, devices need bandwidths three-to-four times higher than the intended operation frequency, which means that if one wants to generate power at 30 GHz, for say, electronic warfare applications, one needs devices with bandwidths in excess of 100 GHz with a lot of power gain at 30 GHz.
Figure 1. An AlInN/GaN HEMT fabricated at ETH-Zurich from EPFL epitaxial layers
Nitride devices also offer high power-added-efficiencies which are, for example, critical in airborne applications because of the limited aircraft payloads. For instance, a high-resolution radar operating between 27 and 40 GHz must radiate maximum power within a fixed power budget.
The inherent ruggedness associated with widegap semiconductors also potentially renders wideband GaN HEMTs attractive for low-noise, front-end amplifiers. It is possible that these amplifiers could be free of the performance-degrading input protection devices used today to protect sensitive GaAs or InP front ends from damage due to over-voltage.
There is currently also research targeting the development of 500 GHz GaN HEMTs with 10 V breakdown voltages for special digital ICs − whether this is a realistic goal remains an open question, but the work clearly inscribes itself under the umbrella of speed at higher power levels, since nowadays such bandwidths can be only reached with devices showing 3-4V breakdown voltages at best, namely InP DHBTs.
What can GaInAs teach us?
Some inspiration on how to extend GaN HEMTs to mmwave frequencies can be gained by considering the history of GaInAs channel HEMTs. Since the late 1980s the cutoff frequency record for the GaInAs-based HEMT has steadily climbed from 100 GHz to in excess of 600 GHz. The refinements that have driven this progress offer insights on how to speed-up GaN HEMTs, because ironically, despite the widely different energy gaps and other physico-chemical properties of the materials involved, a number of interesting parallels can be drawn between the two technologies.
The fantastically successful evolution of GaInAs-based HEMTs has on one hand relied on the development of a superior channel layer. Such a channel can confine higher electron densities, and has the added advantage of reducing parasitic delays − channel charging delay, and drain depletion delay − therefore increasing transistor cutoff frequencies. Stronger carrier confinement in the channels results from an increase in the indium content of this layer, an approach that was initially employed in pHEMTs on GaAs before being extended to InP.
The other key to speeding up GaInAs HEMTs has been the location of the gate electrode with respect to the current carrying channel layer. By placing the gate very close to the two-dimensional electron gas (2DEG) channel, it becomes possible to realize deep-sub-micron devices that maintain a good electrostatic control of the channel electrons by the gate electrode, as evidenced by minimized short-channel effects.
In the world of MOSFETs, engineers would characterize such devices as being "well tempered." By that they mean that the density of channel electrons is well-controlled by the gate electrode, and the channel is effectively shielded from the influence of the drain contact. InP-based materials show weaker surface depletion effects than GaAs, allowing gates to be placed closer to the channel.
Keeping the gate-to-channel distance small compared to the gate length increases device transconductances, and leads to a faster charging and discharging of intrinsic and parasitic capacitances, and ultimately, to faster transistors.
Lattice-matched barriers?
The conventional form of GaN-based HEMT, which involves the pairing of AlGaN and GaN, has undergone more than a decade-and-a-half of frantic development. However, question marks are now hanging over the reliability of this device (see, for example [1, 2]). These are thought to stem from inherent strain in the heterostructure, due to a combination of lattice-mismatch and piezoelectric contributions.
Another concern with this AlGaN/GaN incumbent is surface depletion effects in the 2DEG that arise when the top barrier thickness is thinned much below 15 nm [3]. This can be addressed with AlN thin top barriers that increase channel sheet densities [4], but it is unclear how this impacts the strain-related device degradation. Other research groups have countered surface depletion effects with aluminum-rich AlGaN barriers and various dielectrics, an approach that has yielded impressive cutoff frequencies, with fT values reaching 190 GHz with 60 nm gate MISFET structures [3, 5].
One promising alternative to the AlGaN/GaN HEMT is a variant based on a heterostructure between AlInN and GaN. This potential successor, which was proposed by Jan Kuzmuk from the Technical University of Vienna, can be fabricated with a lattice-matched barrier and thus addresses some of the strain-related reliability concerns associated with conventional lattice-mismatched, AlGaN/GaN heterostructures [6]. Kuzmuk has also argued that the pairing of AlInN and GaN should reduce surface depletion effects and potentially unlock the door to the fabrication of GaN HEMTs with excellent channel aspect ratios down to very short gate lengths.
Figure 2. X-ray analysis showing AlInN compositional uniformity for material grown on (111) silicon
Early experimental efforts suggested that the AlInN/GaN pairing is capable of fulfilling many expectations. The feasibility of ultrathin barrier AlInN/GaN HEMTs has been verified down to 3 nm thick AlInN barriers [7], and such devices have also demonstrated phenomenal stability, even surviving operation at temperatures of 1000 °C [8]. Such high-temperature survival is clearly outside the reach of traditional AlGaN/GaN devices.
To put it simply, it seems that AlInN/GaN heterostructure channels are capable of driving evolutionary improvements reminiscent of those achieved by the transition from GaAs-based pHEMTs to their InP counterparts. And it seems that these benefits can be realized without paying the penalty of more fragile materials, as was the case when moving from GaAs-based HEMTs to high-indiumcontent GaInAs alloys on InP.
One key benefit that results from the weaker surface depletion effects in AlInN/GaN HEMTs is a vertical scaling advantage − this is very similar to that seen for AlInAs/GaInAs HEMTs over AlGaAs/GaInAs equivalents [9]. In addition, HEMTs based on AlInN were reported to exhibit higher carrier velocities in the channel, thanks to faster thermalization and decay of hot longitudinal-optic phonons. The upshot of this is higher electron velocities in strong electric fields [10].
Although the analogy to InP pHEMT development is very good, there is one difference between GaN and InP devices. With InP, higher channel velocities stemmed from improved transport properties in GaInAs alloys with a high indium content, which made the devices more fragile, due to the lower temperature stability of high indium content materials.
In comparison, while the AlInN/GaN system does contain some indium, it is the higher aluminum content that is responsible for the ruggedness advantage that AlInN/GaN HEMTs have over their AlGaN/GaN equivalents. The aluminum content in the AlInN alloy that forms the lattice-matched barrier on GaN is 83 percent. This is far higher than the mere 30 percent in strained AlGaN barriers, a fundamental difference that appears to confer a far greater stability of the newer form of HEMT.
By putting some faith into the analogy between AlInN/GaN HEMTs and InP pHEMTs, it is just a small further step to reach the idea that an AlInN/GaN HEMT should yield superior cutoff frequencies compared to its conventional predecessor - just as the case was for InP pHEMTs compared to GaAs pHEMTs.
However, until recently, AlInN/GaN HEMTs have failed to fulfill this promise, conceding a significant bandwidth advantage to their forerunners. But the good news is that thanks to material and process improvements, the ultimate cutoff frequency of AlInN/GaN HEMTs has more than doubled in the last year [11, 12].
Growing AlInN
At first sight it appears quite challenging to grow highquality AlInN films. There are huge differences in growth conditions of AlN and InN, which have typical growth temperatures of 1100 °C and 500 °C, respectively. In addition, the lattice mismatch between these materials can be as high as 13 percent. However, it is possible to realize a homogeneous ternary through careful optimization of the growth conditions.
At EPFL in Lausanne, Switzerland, AlInN/GaN epilayers are grown in an Aixtron 200/4 RF-S MOCVD system on 2-inch substrates made from c-plane sapphire, silicon, and SiC. Growth on sapphire is initiated by a lowtemperature GaN nucleation layer, and an AlN buffer is employed for silicon and SiC. In all cases, a 0.5-2 μmthick, undoped GaN layer follows the buffer. This is grown using conditions to minimize possible parasitic conduction paths, and its net residual doping concentration (ND-NA) is typically below 1014 cm-3.
All HEMT structures are free from cracks. Dislocation densities in the epilayers are governed by the substrate choice, and range from 7 x 108 cm-2 for sapphire to 5 x 109 cm-2 for silicon. Typical X-ray diffraction (XRD) rocking curve linewidths are less than 1000 arcsec for GaN deposited on silicon, below 500 arcsec for GaN grown on sapphire, and under 200 arcsec for GaN epitaxial layers on SiC.
The AlInN/GaN heterostructure features a thin GaN channel, grown under conditions specifically chosen to improve surface morphology so as to form a good interface with the barrier material. An AlN interlayer just a nanometer thick is inserted between the channel and the AlInN barrier to limit the detrimental impact of alloy scattering. When properly done, this delivers a massive improvement in the 2DEG lateral transport properties. Growth of the AlInN layer is typically carried out at 800- 850°C, using deposition rates of 0.2-0.6 μm/h. If the temperature is too low, the crystal quality degrades, according to high-resolution x-ray diffraction (XRD). On the other hand, if the temperature is too high, it impairs indium incorporation and prevents formation of near-lattice matched alloys. Treading the fine line between these two unwanted scenarios is crucial to realizing good 2DEG properties, and ultimately is the key to great HEMT performance. Material uniformity of thick, nearly lattice-matched AlInN epilayers grown on GaN-onsapphire templates can be assessed by energy dispersive X-ray analysis. The indium composition slowly varies across the wafer indicating good homogeneity (see Figure 2). It is typically 17 1 percent for lattice matching to GaN. The surface of the AlInN barrier is very smooth and has a root-mean-square roughness of just 0.5 nm (see Figure 3).
Figure 3. Atomic force microscopy (AFM) measurements indicate that the AlInN barrier is very smooth. The root-meansquare roughness is just 0.5 nm
Scanning transmission electron microscopy (STEM) and energy-dispersive X-ray spectroscopy (EDXS) analysis reveals a sharp interface, with no evidence of gallium diffusion into the AlInN barrier (see Figure 4) [13]. If this element, gallium, had significantly contaminated the barrier, it would decrease the 2DEG electron density in the channel. By varying the indium content of the AlInN barrier and its thickness, it is possible to realize 2DEG densities ranging from 0.5-3.5 x 1013 cm-2.
Figure 4. Gallium does not diffuse into the AlInN barrier, according to STEM and EDXF analysis. These are reproduced courtesy of Dr. L. Zhou and Prof. D.J. Smith, Arizona State University
Room-temperature mobility in the 2DEG depends on both the sheet carrier density and the type of substrate (see Figure 5). The lowest sheet resistances, typically 200 Ω/are obtained on sapphire and SiC. On silicon, the sheet resistance is slightly larger, due to the lower crystalline quality of epitaxial layers (as revealed by the XRD linewidths). However, resistance is still only 300 Ω/. This value indicates that silicon can provide a platform for realizing high-performance millimeter-wave transistors on low-cost substrates. The thermal conductivity of silicon lies between that of sapphire and SiC, and it has the potential to be used as a low-cost platform for GaN/AlInN HEMTs in either systems requiring lower CW power operation, pulsed output (such as radar) and/or in systems requiring many cheap devices (decoys).
Figure 5. Sapphire and SiC still offer superior platforms to silicon for the growth of AlInN/GaN HEMTs, but silicon is not too far behind
Record breaking devices
Our epitaxial HEMT wafers include a structure with a 30 nm AlN nucleation layer, a 1 μm-thick GaN insulating buffer and channel layer, a 1 nm AlN spacer layer, and a 10 nm thick, nearly lattice-matched Al0.86In0.14N barrier. This structure had a channel electron sheet density of 2.4 x 1013 cm-2, a mobility of 1,300 cm2/Vs, and a sheet resistance of 200 Ω/, according to room-temperature Hall measurements. Incidentally, similar AlInN/GaN HEMT epilayers are commercially available from EPFL start-up NovaGaN.
The layers were processed in the ETH-FIRST Laboratory in Zurich. Mesas were defined in the epiwafers by plasma etching, before ohmic contacts were added by Ti/Al/Au evaporation. Two-step rapid thermal annealing in N2/H2 forming gas followed.
Post-process transmission-line measurements revealed contact and sheet resistances of 0.3 Ω/mm and 182 Ω/. Electron-beam lithography defined Ni/Au T-shaped gates with a 55 nm footprint in the center of the 1 μm source/drain space using high resolution electron beam resists. A 100 nm-thick, SiN passivation film was then deposited by plasma-assisted CVD and patterned to create the contact pads. Ti/Au was used for the overlay metallization. The 55 nm gate HEMT produces a maximum drain current of 2.3 A/mm at a gate-source voltage (VGS) of 0 V (see Figure 6). Extrinsic transconductance (gM ) peaked at 575 mS/mm when the HEMT was operated at a VGS = -5.3 V and VDS = 4.0 V.
Figure 6. Current-voltage characteristics for an AlInN/GaN HEMT grown on semiinsulating SiC. The gate length of the device is 55 nm
AlInN/GaN devices often show a residual gate leakage current, and this is now a topic of investigation in various groups. Understanding the leakage mechanism, and stemming its flow, will be key to increasing the poweradded- efficiency characteristics of these HEMTs, ultimately enabling them to meet the efficiency requirements for certain applications.
The gate leakage origin still is a mystery, but not all researchers involved with AlInN/GaN HEMTs experience excessively high gate leakage currents. Leakage may be primarily associated with epitaxial growth, device processing or even a combination of both. At this time it is known that one can reduce it by inserting insulators between the gate metal and the barrier, but it remains desirable to suppress leakage in a standard HEMT structure to keep the gate as close to the 2DEG as possible. At this point it should be kept in mind that the leakage issue may partly arise due to the thin barriers used − it is then normal to expect a higher leakage than with thicker barriers, just as it is observed in AlGaN/GaN HEMTs!
Transistor microwave performance at the peak fT bias of VDS = 4 V and VGS = -5.3 V has been measured (see Figure 7), and extrapolation of the short-circuit current gain |h21|2 and of Mason's unilateral gain U with a -20 dB/dec roll-off yields a fT of 205 GHz and fMAX(U) of 191 GHz. The fT and fMAX remain high for a broad range of drain biases, remaining above 160 GHz up to VDS of 8 V. Increasing the drain bias to a VDS of 5 V resulted in a peak fMAX(U) of 200 GHz with an fT of 188 GHz at a VGS of -5.3 V. A cutoff frequency of 205 GHz is the highest-ever achieved for any GaN-based transistors on any substrate. It is also the first time that AlInN/GaN-based devices have established the record for bandwidth in nitride HEMTs. Although one can expect records to continue to change hands between different types of GaN HEMTs, these results are extremely pleasing considering the rapidity of progress with the new AlInN/GaN heterostructures.
Figure 7. The AlInN/GaN HEMTs fabricated in the EPFL-ETHZ collaboration deliver a record breaking cut-off frequency for any form of GaN HEMT
Analysis of the frequency performance, which will be published shortly, reveals that the effective velocity in AlInN/GaN channels is up to 60% higher than in the fastest AlGaN/GaN HEMT channels ever produced. Some theoretical and experimental work has linked higher channel velocities in AlInN/GaN channels to the higher 2DEG concentrations. These are believed to favor the faster dissipation of longitudinal optical phonons in comparison to the situation in conventional AlGaN/GaN channels. Today, however, it is not possible to fully explain the improved performance observed with AlInN/GaN by this phenomenon. Further studies are necessary. For the sake of comparison, AlInN/AlN/GaN HEMTs were fabricated in a similar manner using epilayers grown on high-resistivity silicon (111) substrates. In this case, the epitaxial structure comprised a 60 nm AlN nucleation layer, 700 nm GaN insulating buffer and channel layer, a 1 nm AlN spacer layer, and an 8 nm-thick, nearly latticematched, Al0.86In0.14N barrier. According to roomtemperature Hall measurements, the channel electron sheet density was 1.95 x 1013 cm-2 and its mobility was 1,060 cm2/Vs. These inferior values compared to the above layers on SiC are a result of a reduction in crystal quality, which was highlighted by broader XRD linewidths. The 2DEG and mobility are 20-25 percent lower than that of the AlInN/GaN HEMT on SiC, and account for the increase in sheet resistance to 300 Ω/.
Devices with a 80 nm gate length were fabricated on these layers, which show simultaneous cutoff frequencies, fT of 143 GHz and fMAX(U) of 176 GHz. Although these values are lower than those achieved with the 55 nm devices on SiC, they are still the fastest nitride devices ever built on silicon. It is also interesting to note that the fT's obtained here for the 55 and 80 nm gate HEMTs on SiC and silicon substrates scale almost exactly with the inverse gate length. Clearly, the AlInN/GaN heterostructure has more surprises in store than one could ever anticipate!
AlInN/GaN HEMTs are fast emerging as an interesting alternative to conventional AlGaN/GaN HEMTs which offers much higher maximum current densities and transconductances in a (nearly) lattice-matched barrier system characterized by a superior thermal stability. Although the present article mainly focused on recent EPFL/ETHZ efforts toward extending the devices to higher frequencies, the fundamental properties of the AlInN/GaN system do lend themselves well to the realization of highpower, high-efficiency transistors, as recently reported by workers from Alcatel-Thales with the demonstration of 0.25 μm AlInN/GaN HEMTs with an output power of 10.3 W/mm and a power-added efficiency of 51% at 10 GHz [14]. The combined advantages of AlInN/GaN heterostructures for power HEMTs are extremely attractive and provide ample motivation to find solutions to remaining challenges associated with the material system: one can count on an increasingly important footprint for AlInN/GaN HEMTs in the field of GaN microwave and millimeter-wave electronics for the times to come.
References
1. S.Y. Park et al. Microelectronics Rel. 49 478-483 (2009)
2. A. Chini et al. Electron. Lett. 45 426-427 (2009)
3. M. Higashiwaki et al. Proc. SPIE 6894 68941L (2008)
4. Y. Cao et al. Appl. Phys. Lett. 90 182112 (2007)
5. M. Higashiwaki et al. Appl. Phys. Express 1 021103 (2008)
6. J. Kuzmuk IEEE Electron Device Lett. 22 510-512 (2001)
7. F. Medjdoub et al. IEEE Electron Device Lett. 29 422-425 (2008)
8. F. Medjdoub et al. The Open Electrical and Electronic Engineering Journal 2 1-7 (2008)
9. L.D. Nguyen et al. IEEE Proc. 80 494-518 (1992)
10. L. Ardaravicius et al. J. Appl. Phys. 106 073708 (2009)
11. H.F. Sun et al. IEEE Electron Device Lett. 30 796-798 (2009)
12. H.F. Sun et al., IEEE Electron Device Lett. 31 293-295 (2010)
13. L. Zhou et al. Appl. Phys. Lett. 94 121909 (2009)
14. N. Sarazin et al. IEEE Electron Device Lett. 31 11-13 (2010)