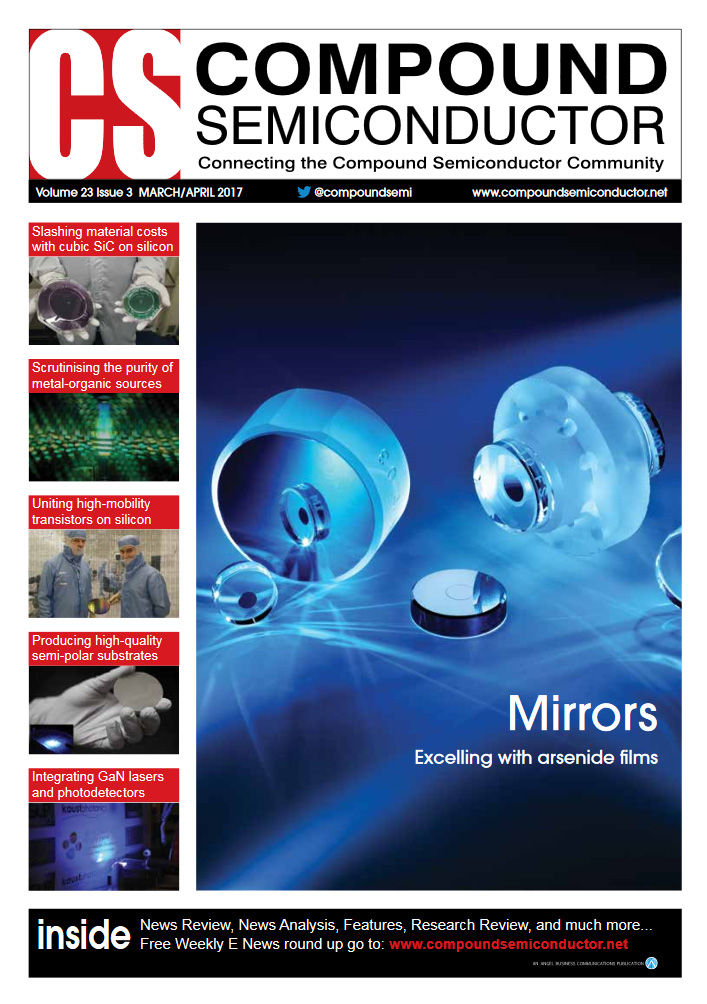
Evaluating the capability of UV LEDs
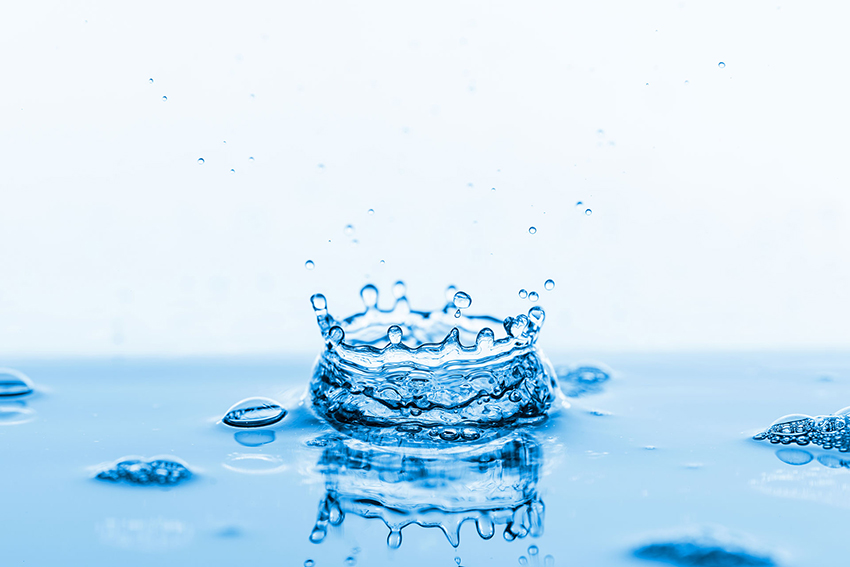
A true appreciation of the performance of a UVC LED requires an understanding of its temperature dependent output power and its spectral profile
BY LEO SCHOWALTER FROM CRYSTAL IS
There are critical consequences to inadequate disinfection of drinking water and medical surfaces. Consider these two statistics: one in ten people still have no access to clean drinking water; and one in every 25 patients acquires an infection while hospitalized, causing healthcare costs to escalate by billions of dollars.
Fortunately, a revolution is underway, and new solutions are modernizing the way disinfection is being accomplished with greater design flexibility and cost effectiveness. A new generation of products is appearing that are equipped with powerful LEDs that emit in the UVC band, which spans 200 nm to 280 nm. Armed with these solid-state sources, more compact designs can be introduced in everything from portable systems for drinking water purification to hospital infrastructure that combats airborne infections.
Figure 1. A very simple model for the temperature rise in a typical Crystal IS Klaran diode as a function of pulse length. Note that Klaran UVC LEDs are manufactured on native AlN substrates, so will heat up very quickly and (nearly) uniformly, due to the high thermal conductivity of AlN. While generally this model applies to all UVC LEDs, diodes fabricated on Al2O3 have an order of magnitude lower thermal conductivity, and may have more temperature variation across the chip at higher powers.
With adoption of UVC LEDs on the rise, the number of manufacturers offering these devices is increasing, and there is more interest than ever in quantifying the performance of the light source. Key performance characteristics in these applications are output power and germicidal effectiveness.
Power output factors
With all types of LEDs, regardless of their emission wavelength, light is emitted out of the front of the device, while heat is extracted from the back. During operation, as the junction temperature increases, the LED's light output decreases.
With a visible LED, typically 40 percent to 60 percent of the electrical energy is converted to heat. That is considerable, but in the case of UVC LEDs, 95 percent or more of the energy is converted to heat. Therefore, it is critical to properly manage the heat to maintain light output. This concern is amplified by the market drive to decrease LED footprint, resulting in compact packages, which limit built-in heat absorption or dissipation design options.
To appreciate the importance of good thermal management, note that if a UVC LED has not been mounted to a heat management system, it can reach 200°C in less than a second. At this temperature, the device output will drop to near zero and have a very short lifetime.
For customers seeking to characterize diodes without soldering them to a thermal management system, a pulse measurement approach is recommended to limit heat generation within the device and provide reliable, repeatable output power measurements. This is the same process Crystal IS and other manufacturers are adopting to measure UVC LED output as these devices are commercialized.
Figure 2. Crystal IS measures Klaran device power by waiting 3 ms after the start of the current pulse and integrating the output power for 8 ms.
We can use a very simple model for the temperature rise in a typical Crystal IS Klaran diode as a function of pulse length or duration (Figure 1). Heat is carried away from the chip by thermal conduction of the gold bump bonds used to attach it to the rest of the LED package. Because the thermal resistance within the chip is much smaller than the bonds and the heat capacity of the chip is much smaller that the heat capacity of the package, the chip temperature will rise rapidly. This will continue until the temperature difference between the chip and the package is approximately equal to the product of the input power times the thermal resistance, at which point the heat generated in the chip will be approximately equal to the heat carried away by thermal conduction.
Following this initial hike in temperature, the junction temperature rises more slowly as the package heats up. After a few milliseconds, the slope of the temperature rise approaches the power in, divided by the capacity of the package. The temperature continues to rise until a significant amount of heat can flow into the ambient environment.
After the first few milliseconds of operation of the LED, keeping the package cool requires heat to flow away from the package to the heat sink (this is illustrated in Figure 1). When this happens, the thermal resistance between the package and the thermal management system is determined by the combination of the quality of the solder to the heat sink, and the ability of the heat sink to either absorb energy, or radiate it to the environment.
If the device is operated without a heat sink, the junction temperature will continue to rise, which will lead to a decrease in light output. Nonetheless, using a pulse mode can still carry out an accurate output power characterization of the diode. To improve accuracy and reproducibility, the power output should be measured during the gradual increase portion of the curve, not in the steep incline of the first millisecond. This allows the temperature difference between the chip and the package to stabilize at approximately the same value it will have between the LED chip and solder pads. In addition, the power measurement should be taken at the shortest time possible "“ just enough to capture the diode's power output while minimizing the package's heat induced temperature rise. We measure devices by waiting 3 milliseconds (ms) after the start of the current pulse and then integrate the output for 8 ms, which means we stop integrating 11 ms after the start of the pulse (Figure 2).
Figure 3. Spectral comparison of low and medium pressure mercury lamps versus LED in relation to typical DNA absorption curve.
There are pitfalls associated with the failure to appreciate the influence of the measurement time when attempting to determine the output power of a UVC LED. If we take an example using a Crystal IS Klaran LED with a specified output power of 20 mW operated at an ambient temperature of 25°C. The heat capacity of the package is approximately 20 mJ/K, which means the slope for the temperature rise is approximately 200 K/s for a 4 W input power. As this diode has a specified thermal derating of 0.5 percent per Kelvin, power will fall by 5 percent if the junction temperature increases by 10°C.
We then assess the output power of our LED by driving it with an 80 ms pulse, and measuring the output power after 40 ms for 40 ms. During this process, the chip's temperature has an initial jump in the first millisecond, due to heating. After that, within the first 40 ms the junction temperature increases an additional 8°C "“ that's 0.2°C per millisecond "“ with respect to the package. Once 80 ms has elapsed, which occurs following another 40 ms of the diode being run without a heat sink, the junction temperature increases another 8°C. During the first 40 ms, the instantaneous power of the diode drops by 4 percent, and it falls by this amount again in the next 40 ms. So, assuming that measured power is obtained by averaging the power between 40 ms and 80 ms, the measured decrease in power is 6 percent.
In practical terms, this average is obtained by integrating the power for 40 ms, and dividing the measured energy detected by that integration time. This gives an integrated power is 18.8 mW, which is a 6 percent reduction of the 20 mW specified power. This difference in the measured output, compared with that specified on the data sheet, highlights the impact of the increased junction temperature.
Unique challenges
UVC LEDs are specified to run at a maximum input power and operate within a pre-determined design temperature range. These design specifications are outlined on the diode's product data sheet and manufacturers calibrate their testing equipment to ensure the product shipped to customers meets these specifications. To ensure component quality, customer quality control engineers should test/measure an LED like the OEM. In the previous example, an integrating sphere was used to measure the total power output of the Klaran diode during pulse mode.
Figure 4. Action spectra of common target/challenge microbes in water disinfection normalized to 1 at 265 nm. The action spectrum of B. Subtillis as defined by the ÖNORM Standard; E. coli as outlined in A review of UV lamps by Henk F. J. I. Giller, in WEF 2000; and MS2 as found in This Way Forward: Addressing Action Spectra Bias Concerns In Medium Pressure UV Reactors, Bryan Townsend, et al.
Manufacturers adopt one of two methods for measuring the light source's radiant flux, often noted as the device power. They either use an integrating sphere, which collects all the light from a source placed inside the sphere; or they turn to a goniophotometer, which records the radiant flux of a light source from many different angles around the source to yield a radiant flux as a function of angle. Armed with the later, it is possible to calculate the radiant per solid angle, which is called the radiant intensity "“ integrating this over all angles yields the radiant flux for the light source.
When measuring power output with a goniometer, it is important to resist the urge to assume a particular radiation pattern. It is tempting to save time by measuring the radiant intensity for one LED, and then, after recording the power of subsequent diodes at one fixed angle, use this single value to infer the total power of the diode. This is flawed when the radiant intensity varies between devices, due to differences in packages or manufacturers. The use of integrating spheres avoids this issue, because they are designed to accurately measure the power emitted by the diode, regardless of the radiation pattern.
Determining germicidal power
Knowing an accurate value for the power output of a UVC LED does not, in itself, reveal the capability of this source for a disinfection system. That's because the effectiveness of this radiation is wavelength dependent, with light in the range of 250 nm to 280 nm most effective at inactivating the DNA of microorganisms, rendering them unable to reproduce.
Historically, disinfection has been accomplished by low and medium pressure mercury arc lamps. Low pressure lamps emit a single wavelength of 253.7 nm, so the total output power of the lamp is equivalent to the output power in the UVC range, albeit at a sub-optimal wavelength. Medium pressure lamps, in contrast, emit a broader spectrum, including wavelengths in the germicidal range, plus those that do not contribute to disinfection. With these lamps, typically just 20 to 30 percent of the light is emitted in the UVC range.
With UV LEDs, the spectral profile is much more suitable (see Figure 3). The continuous spectral response of these sources deliver greater overlap with the most critical wavelengths for disinfection, making them more efficient light sources for these systems. However, these differences in emission spectra require a new methodology to account for disinfection effectiveness.
Figure 5. Ratio of total power to germicidal power, based on the ÖNORM standard for a range of commercially available UVC LEDs. Even minor changes in wavelength have a significant impact on the germicidal power available. This is a particular problem at longer wavelengths, where the efficacy of the radiation is rapidly changing with wavelength.
It's a situation that is very similar to the one faced by makers of visible light sources. They measure brightness with the lumen, a unit that takes into account the spectral response of the human eye. For UVC LEDs, what matters is the power output that inactivates pathogens. This is known as the "˜germicidal power'.
The most accurate method to specify the required germicidal power for a particular application is to know both the specific pathogen to be inactivated, and its action spectrum "“ that is, this pathogen's unique profile of sensitivity, by wavelength, to a light-source. Once that is known, the germicidal power of the UVC LED can be determined by the cross product of the light source and the action spectrum for the pathogen.
Different pathogens have different levels of susceptibility to UVC energy (see Figure 4 for an example). Although all peaks close are to 265 nm, the peak absorption wavelength for DNA, there are variations in the sensitivity to discrete wavelengths (see Table). Multiplying the emission of UVC diodes by a weighting determines the power output, in terms of the power available for the disinfection of a specific pathogen.
As applications for UVC LEDs expand, the number of providers will increase. This has pros and cons for OEMs, who have greater choice, but must contend with greater variation between product specifications. When an engineer designs and develops a product, they may prefer to observe the spectrum of an individual light source, so that they can determine the optimum benchmark performance criteria. But high-volume manufacturers desire a more systematic approach for specifying germicidal output power. This is possible with convolution "“ that is, the normalizing of LED output in terms of germicidal power.
We have evaluated the performance of UVC LEDs from a number of manufacturers, by plotting the ratio of germicidal-to-total power, as a function of wavelength (see Figure 5). This reveals that when the total diode power output is the same for all providers, there is still a significant variation in germicidal power, due to differences in peak wavelength. For example, a 285 nm LED from one supplier can have the same total power as another at 265 nm, but only 40-50 percent of the germicidal effect.
Also note that there is an inconsistency in germicidal effect as diodes stray away from the peak absorption of DNA (see Figure 5). This is important, for it could mean the difference between meeting the dosage requirements for safe drinking water, and failing to do so, which would leave active pathogens to infect customers.
While complex microbiological systems have no single approach that fits all needs, specifying light sources based on germicidal powers does offer a step forward in simplification, allowing engineers to create reasonable designs for manufacturability. These sources combine a higher efficiency in germicidal wavelengths with a compact footprint, and can produce a reduction of more than 99.99 percent in pathogens, giving them proven effectiveness in water disinfection. In the coming years, there is no doubt that there will be an uptake in UVC LEDs for disinfection.