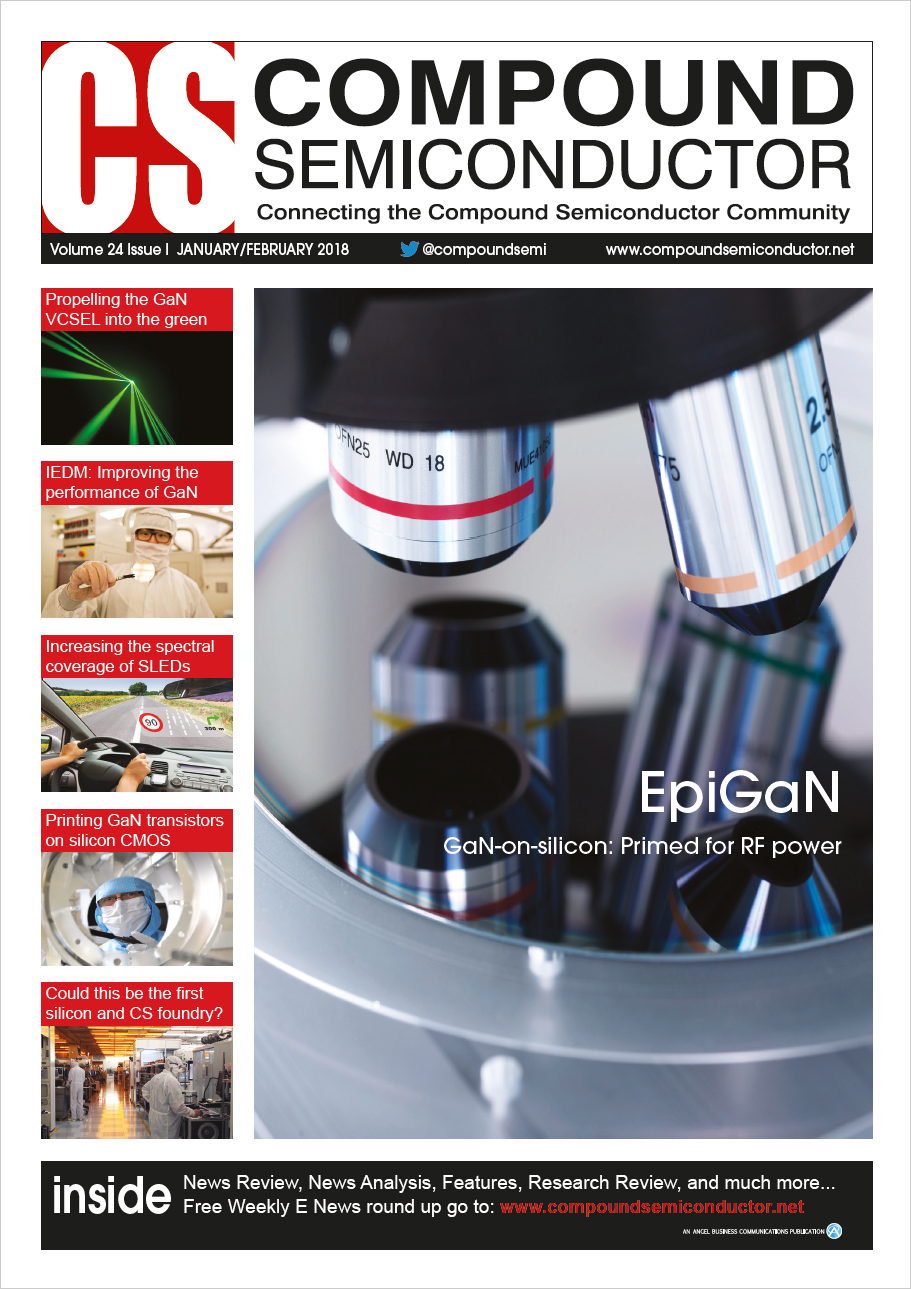
Superluminescent LEDs target displays
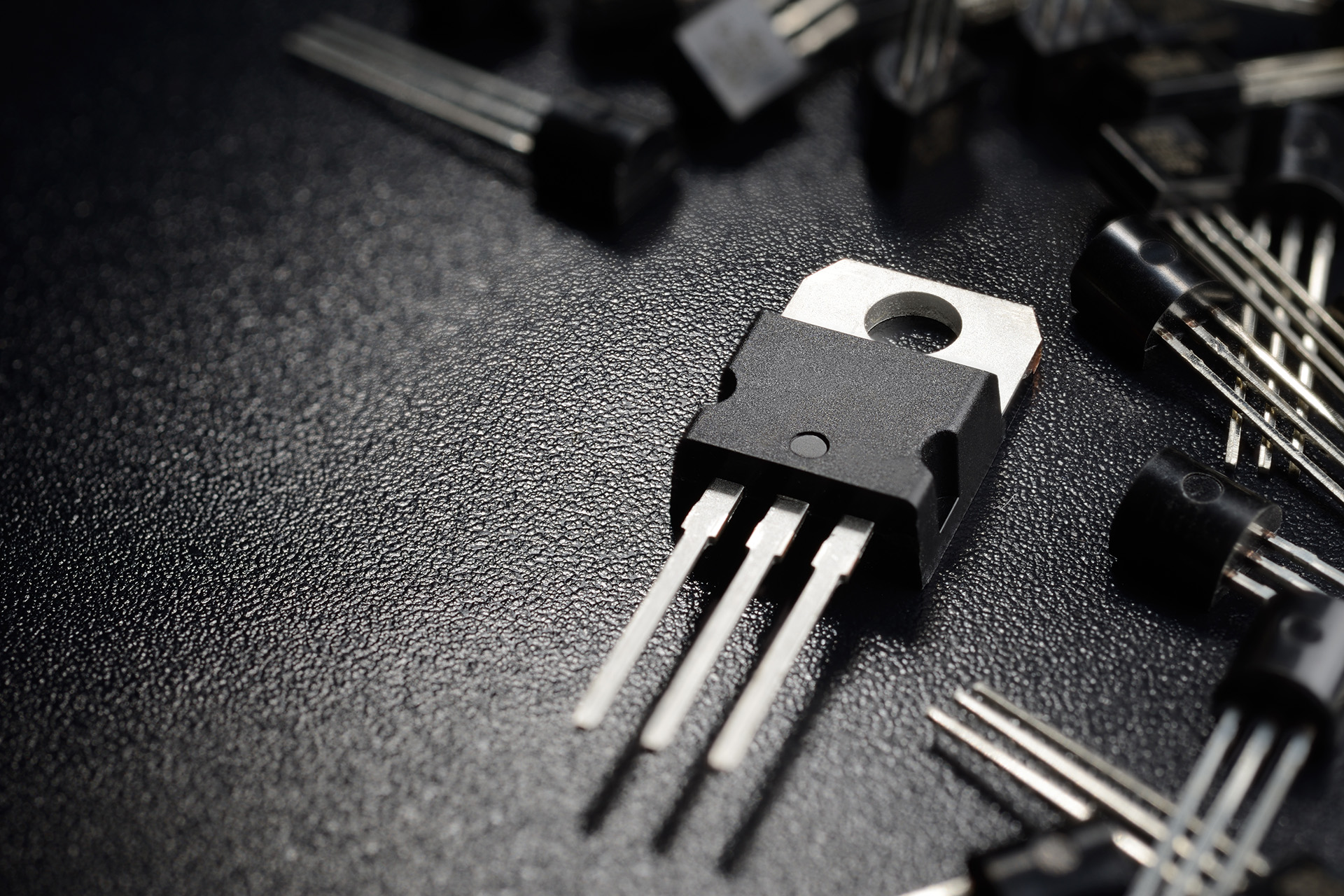
Superluminescent LEDs with record-breaking output powers and
efficiencies underscore the promise of these devices for head-up
displays and pico-projectors by Marco Malinverni from Exalos
One of the hottest research topics right now is the development of energy-efficient display technologies for augmented-reality head-up displays and pico-projectors.
Today, these technologies tend to use laser diodes or LEDs for their light sources "“ but neither is ideal. LEDs are held back by their Lambertian emission profile that translates into low directionality, which hampers coupling to optical elements, such as fibres and waveguides. Laser diodes are better in this regard, because they emit a narrow beam of light that enables simple, efficient coupling to optical elements. What's more, they have a reduced output dimension, so are much closer to an ideal point-like source, aiding optical system design. However, their monochromatic spectral emission, arising from the Fabry-Pérot cavity that is required for optical feedback, results in a high degree of temporal coherence. This is undesirable: when the laser is used to form an image, it is plagued by an interference pattern, commonly referred to as speckle. This is a particularly troublesome for augmented-reality applications, where high-fidelity images are critical. Despeckling solutions have been developed to minimize interference. But success is limited, and the hardware that is required is often too bulky or expensive to incorporate into consumer electronic devices.
What's needed is a source that combines the best attributes of the laser and the LED. And the good news is that this device already exists, in the form of a superluminescent LED (SLED). It provides a directional light source with a broadband emission spectrum that yields a low degree of speckle noise and a high image quality (see Figure 1).
We are pioneering SLEDs at Exalos, Switzerland. One of our milestones came in 2009, when after three years of development, we introduced the industry's first blue-violet (420 nm) SLED in collaboration with the Ecole Polytechnique Fédérale de Lausanne. Fast-forward to today and we are still the only manufacturer of violet and blue SLEDs. That's not our only product, however "“ we are also the leading supplier of a wide range of infrared SLED devices, having shipped more than 300,000 devices for use in a diverse range of applications, from current sensing to fibre-optic gyroscopes and optical coherence tomography.
Recently, we have focused our efforts on increasing the emission wavelength of our SLEDs. If blue and green devices, made from the III-nitride material system, can be paired with red SLEDs based upon more well-characterized GaAs structures, then this triumvirate can be used to form a full-colour display. There is much work to do, though, as the performance of blue SLEDs can still be improved, while those in the green are still in their infancy.
The SLED design
All our SLEDs share the edge-emitting, ridge-waveguide architecture of the laser diode. However, to prevent optical feedback and lasing, cavity effects are limited by increasing mirror losses at the output facet. There are several options for realizing this: tilting the output facet by several degrees, applying an anti-reflection coating to this facet, or adopting a combination of the two. By inhibiting the laser action, a directional, broadband emission beam is realized through the stimulated emission of carriers. The spectral bandwidth is far broader than that of a laser, and governed by the gain characteristics of the semiconductor material.
Figure 1. The speckle noise in the far-field pattern of a blue laser diode (left) is far higher than that produced by an SLED (right).
Our efforts at developing blue and green SLEDs are building on the success we had in 2015 with 405 nm devices. Back then we demonstrated a continuous-wave, high-power, single-mode 405 nm SLED that delivered a record output power of 350 mW at room-temperature under a drive current of 500 mA. The ex-facet optical powers falls to 20 mW, while the associated bandwidth, full width at half maximum, is 3.5 nm, when the current is reduced to 100 mA and the device operated under the same conditions.
Another attribute of those devices from 2015 is their low forward voltage: it's just 5 V at 100 mA. We accomplished this by optimising the magnesium doping levels in the p-type layers. The presence of magnesium impacts the electro-optical characteristics of the device and its reliability, but by fine-tuning the magnesium, we produced continuous"“wave, 10 mW SLEDs with a projected lifetime of about 5000 hours (for a case temperature of 25 °C, and applying a failure criterion of a 35 percent increase in drive current).
Figure 2. Fluorescence images of InGaN active regions with p-doped layers show that growth at lower temperatures does not have to lead to a decrease in material quality.
With all III-nitride-based devices "“ and laser diodes in particular "“ there are technical and historical reasons why violet emitters emerged first, followed by blue and eventually green variants. Historically, development focused on 405 nm violet laser diodes, because the short wavelength allowed an increase in optical data storage and was selected as the Blu Ray standard. More recently, interest has grown in blue and green lasers, due to their suitability for displays.
Shifting the emission wavelength of any III-nitride emitter from the violet to the blue or green is far from trivial. There are several challenges associated with realising sufficient crystal quality within the light-emitting layers, and finding a suitable combination of compounds for creating efficient waveguide structures.
Better blues
At the heart of all III-nitride emitters are active regions with InGaN quantum wells sandwiched between GaN barriers. To increase the emission wavelength, more indium must be added to the quantum well. The indium content is moderate in a blue-emitting well, and high in one emitting in the green.
Figure 3. (Left) Refractive index evolution as a function of wavelength for GaN, In0.03Ga0.97N and Al0.06Ga0.94N. (Centre) Refractive index contrast evolution for GaN/AlGaN, and InGaN/AlGaN waveguide/claddi ng combinations. (Right) Far-field pattern for the two different architectures showing strong substrate leakage for the GaN/AlGaN architecture.
Adding more indium to a quantum well has its downsides. Lattice mismatch to other layers increases, dragging down device performance and trimming the critical thickness of the well, which in turn reduces gain. More indium also means more defects, and a higher electric field in the active region. If growth of the device is undertaken along the polar direction (c-axis), these fields can pull apart electrons and holes, impairing radiative efficiency. What's more, to incorporate more indium in the wells, the epitaxial growth is performed at a lower temperature "“ and this can result in the degradation of crystal quality, and also unwanted contamination.
Another issue is that as the indium content is increased in the quantum wells, there is a fall in the thermal stability of the active region. After the active region is grown in a SLED structure (and also a laser diode), the growth temperature is raised for the deposition of the p-type waveguide and cladding layers. The higher temperature can degrade the wells. While growth temperatures for the p-type layers of typically above 1000°C are suitable for the production of 405 nm devices, they degrade the performance of blue and green emitters.
Figure 4. (Left) Lightcurrent-voltage characteristics and wall plug efficiency of Exalos blue SLEDs under continuouswave operation. Emission spectra at 200 mA (right).
To address this particular issue, we have worked on reducing the temperature for the growth of the p-type layer while ensuring that it still provides good electrical conduction. Lowering the growth temperature has its drawbacks, including reductions in both the incorporation efficiency of the p-type dopant, magnesium, and the conductivity of the p-layers. However, there is a sweet-spot below 900°C, where p-type waveguide and cladding layers with good electrical characteristics can be grown that do not lead to the creation of extended defects in the quantum wells (see Figure 2). This growth condition is suitable for both blue and green SLEDs.
Unlike the laser, the SLED relies on single-pass amplification of light. The output produced by this device depends on the material properties of the active region, and also on the light-guiding effects that are related to the architecture.
One way to improve performance is to increase the optical confinement within the device. This is accomplished by cladding the active region and the waveguide with a lower refractive index material, typically AlGaN. However, when moving from violet to longer wavelengths, the difference between the refractive index of this cladding and the waveguide diminishes, leading to a lower optical confinement and a compromised output power (see Figure 3). This means that the traditional combination of a GaN waveguide and an AlGaN cladding, used in violet devices, is unsuitable for blue and green devices.
Our solution is to switch from GaN waveguides to those made from InGaN. To determine the best design, we have undertaken extensive simulations on waveguide-cladding architectures, uncovering ideal layer thicknesses and compositions. This effort has also led to an improvement in output beam quality, due to a dramatic reduction in the leakage of light towards the substrate. To realise this benefit, we had to increase the material quality of InGaN, so that this could guarantee low optical and carrier losses in the waveguide. Note that we still use AlGaN in our devices, in the cladding layers. In fact, the bottom cladding is a much thicker layer than it would be in a violet device, in order to reduce the leakage of the optical mode to the substrate.
One of the challenges of incorporating AlGaN into the device is that it has a smaller lattice parameter than GaN, so when this ternary is grown on high-quality GaN freestanding substrates used for laser diode and SLED epitaxy, tensile strain builds up in this layer. Consequently, strain management is critical to prevent cracking in the thick AlGaN layer needed to reduce optical leakage. By applying strain release technologies, we can manage the strain so successfully that we can double the thickness of the AlGaN bottom cladding layer.
Optimising devices
One of the similarities between the SLED and the laser diode is the areas of focus for optimising performance: both devices require low optical losses, high injection efficiency, and high gain. However, gain-guided laser diode processing is extremely rapid, while single-mode SLED processing is complicated and time consuming. So, to speed development, we have processed our epiwafers into both SLEDs and gain-guided laser diodes. The latter device provides fast feedback on the lasing characteristics of the quantum-well structure, such as laser threshold and slope efficiency. By varying the reflectivity of the facet coating, it is also possible to uncover internal device parameters, such as optical losses, modal gain, and carrier injection efficiency.
Using this approach we have developed and optimised blue laser diodes and SLEDs with operating wavelengths of more than 440 nm. Initially, the threshold current densities in uncoated gain-guided laser diodes were as high as 7 kA cm-2. However, these fell to below 3 kA cm-2 after we addressed numerous challenges related to optical confinement, active region stability, and the more-general crystal quality of the layers.
These refinements also led to an increase in slope efficiency, with output from a single facet jumping from 0.3 W/A to more than 0.6 W/A for uncoated devices. Turning to a high-reflectivity rear-facet coating and an uncoated front facet provided further improvement, with the slope efficiency exceeding 1.2 W/A in the latest gain-guided devices. Even lower threshold currents are possible by partially coating the front facet. This enabled threshold currents below 1 kA cm-2.
Using the variable facet coating method, we observed a constant decrease in internal losses during the epitaxial development. Improvements in material quality resulted in a 50 percent reduction in these losses, from above 12 cm-1 to below 6 cm-1.
Figure 5. Exalos demonstrator presented at Display Week 2017 comparing red-green-blue laser diodes (top row) to Exalos red and blue SLEDs (bottom row).
We have processed our optimized epiwafers into single-mode SLEDs. Housed in a TO-can and driven in continuous-wave mode at room-temperature, these blue-emitting devices have current-voltage characteristics that are comparable to 405 nm devices "“ voltages are only few tenths of a volt higher, despite growing the p-doped layers at far lower temperatures.
The ex-facet output powers for our SLEDs exponentially increase above 60 mA. This is encouraging, because it is a typical signature for the appearance of gain in the structure. When the current is increased to 120 mA and then on to 200 mA, the output power rises to 5 mW and 30 mW, respectively, with emission centred at 442 nm. Even higher output powers are possible "“ the maximum is just short of 200 mW without any specially designed heat sink. These powers levels, along with wall plug efficiencies of more than 5 percent, are the highest reported values for any blue SLEDs.
As the next wave of consumer devices for augmented reality applications evolves, the various, competing display architectures will be evaluated. The SLED will surely be a leading candidate, thanks to its unique spectral and spatial properties that give it compelling advantages as an illumination source, especially for near-to-eye architectures.
Our recent results reveal that considerable progress has been made in the development of blue SLED devices. However, further improvement is required for mainstream adoption. We are on track to delivering higher-efficiency devices at 450 nm, and will strive to extend the emission to longer wavelengths. Once emission exceeds 510 nm, SLEDs can provide the ultimate source for red-green-blue illumination.