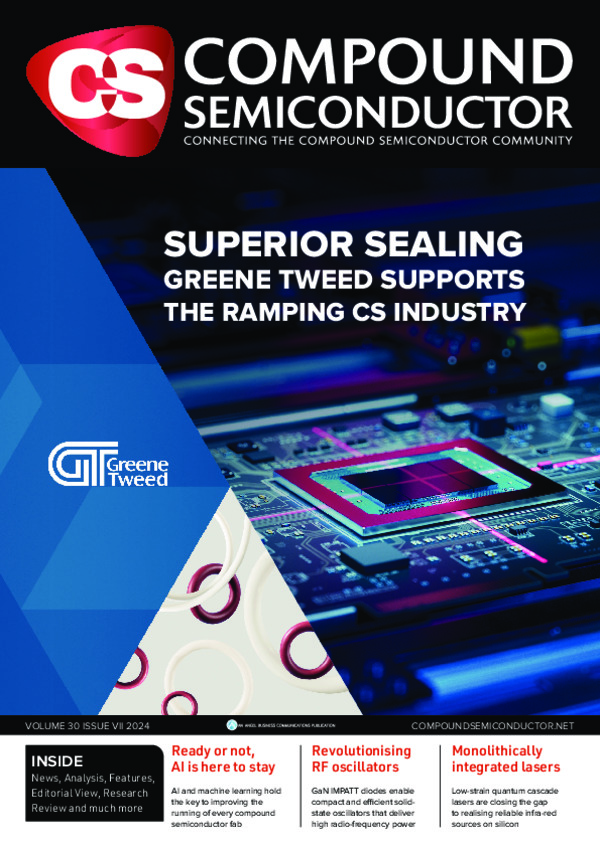
Propelling PCSEL emission to the mid-infrared
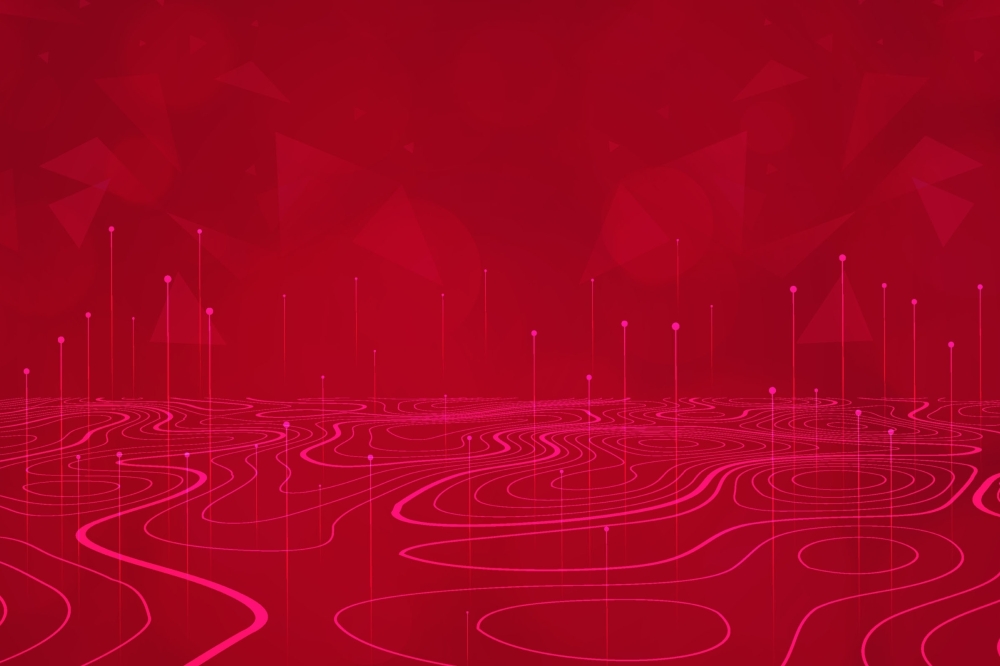
Turning to antimonides extends the emission of the PCSEL to the mid-infrared, enabling this source to provide a new class of cost-efficient active optical sensors.
BY LEON SHTERENGAS FROM STONY BROOK UNIVERSITY
One of the key strengths of the photonic crystal surface-emitting laser (PCSEL) is its generation of a high-power beam with ultra-low divergence and a narrow spectral profile. As this goes hand-in-hand with potentially relaxed fabrication and packaging requirements, the PCSEL makes a compelling case for the ultimate format of semiconductor laser in many applications.
The PCSEL’s advantage originates from the incorporation of a layer that produces a periodic modulation of the dielectric function. Introducing this photonic crystal layer within the laser’s heterostructure transforms its output from that of a multi-mode edge emitter to a single-mode surface emitter. When watt-class output power levels are not required, PCSEL postgrowth fabrication and packaging is virtually identical to that of inexpensive LEDs, opening the door to cost-efficient laser-based sensor systems.
Figure 1. Top of the image shows the band diagram under flat band
condition and corresponding calculated refractive index profile and
intensity distributions of the fundamental mode of the 2 µm PCSEL
device. The bottom of the figure shows the cross-section SEM image of
the buried high-index-contrast photonic crystal layer of the fully
fabricated PCSEL.
Introducing antimonides
A critical technological step associated with PCSEL development is integration of the photonic crystal layer into the laser’s heterostructure. The development of this new class of laser began with some of the more established material systems, such as those based on the arsenides. That’s the material system employed by scientists at Kyoto University to pioneer their air-pocket-retaining epitaxial regrowth.
Turning to antimonide-based materials to realise PCSELs emitting in the mid-infrared is challenging, because epitaxial regrowth within the III-V-Sb material system is not that well developed. Breaking new ground on this front is our research group at Stony Brook University, with successes including the realisation of continuous-wave, room-temperature GaSb-based PCSELs emitting near 2 µm in 2023.
While GaSb-based interband semiconductor lasers are not as common as those based on either GaAs, InP or GaN, they are certainly not in their infancy, and can operate in the continuous-wave regime at room temperature over a wide spectral region that spans from below 2 µm to over 5 µm.
Here we discuss devices based on the so-called type-I quantum-well active region. GaSb-based edge emitting diode and cascade diode lasers with this form of active region operate very well from about 1.9 µm to about 3.3 µm. Note that it is possible to transfer the air-hole retaining regrowth technology that we developed for this class of devices to type-II quantum well interband cascade lasers, which are capable of delivering a strong performance at even longer wavelengths.
We undertake the growth of antimonide-based PCSEL heterostructures using two epitaxial steps. The first, accomplished via MBE, is the growth of an incomplete laser heterostructure that is missing the top cladding layers. The heterostructure that’s formed comprises AlGaAsSb n-cladding, an active region containing GaInAsSb quantum wells embedded into an AlGaAsSb waveguide core, and a thick GaSb top layer.
After removing this incomplete laser heterostructure from our MBE reactor, we use electron-beam lithography to form a hard mask for dry etching our photonic-crystal pattern. Inductively coupled plasma-reactive ion etching defines a square lattice of holes in the top GaSb layer.
Our next step begins by preparing the nano-patterned GaSb surface for regrowth. We apply an atomic-hydrogen-assisted surface-preparation process to clean the surface and remove a native oxide in high vacuum without degrading the crystalline quality of the active region. Regrowth of a nanopatterned incomplete laser heterostructure follows, using AlGaAsSb p-cladding and p-contact layers (see Figure 1 for the band diagram of the regrown diode laser heterostructure as well as calculated refractive index and modal intensity distributions).
Cross-sectional scanning electron microscopy of our regrown wafer containing a buried high-index-contrast photonic crystal layer reveals that a thin layer of AlGaAsSb material covers the etched GaSb surface, even inside the etched holes (see Figure 1). Buried voids are formed, due to self-shadowing of the etched holes from atomic fluxes incoming at the angle to surface normal. Due to this, we are able to integrate a high-index-contrast photonic-crystal layer within a diode laser heterostructure.
Figure 2. Top views of the hard masks fabricated on SiN layer covering
incomplete PCSEL heterostructure. On the left is the mask used to
fabricate PCSELs with single void per unit cell, while on the right is
the mask used to fabricate four-void per unit cell buried photonic
crystal devices. The period of square lattice is 540 nm in both cases.
Challenges and outlook
So far, we have fabricated antimonide-based diode and cascade diode PCSELs operating at up to 2.8 µm. For the diode PCSELs operating near 2 µm, we have demonstrated devices with a continuous-wave output power of 30 mW. Far higher output powers will follow when we increase the outcoupling efficiency of our mid-infrared PCSELs. This is one of our objectives, along with improving the stability of our PCSEL cavities against excitation of the higher-order spatial modes. Our goal is to develop a mid-infrared PCSEL cavity design that delivers an optimal balance between the coupling of the laser modes to active quantum wells and to the buried photonic-crystal layer.
To increase the operating wavelength of a PCSEL, there needs to be a nearly proportional increase in the period of the buried photonic crystal. Realising this is not easy, as it is challenging to scale up the volume of buried voids, because they are not fully controlled by the lateral size of the etched holes – instead, they are strongly influenced by the regrowth step. If the relative size of the buried voids decreases with respect to period of the photonic crystal – a situation that reduces the void area fill-factor – this can result in an undesired reduction of the interaction strength between the laser mode and the buried photonic-crystal layer. The implications are problematic, such as weaker feedback and lower outcoupling efficiencies.
When increasing the period, turning to several voids per unit cell is an attractive option for ensuring an adequate area fill-factor of the void in the unit cell of the buried photonic crystal. This approach has already been adopted with arsenide-based PCSEL technology, where it has been used to fine-tune the buried photonic crystal spatial optical-mode selectivity and promote a high degree of coherence over large emitting apertures. We intend to explore this opportunity very soon. So far, we have studied designs with multiple voids per unit cell, solely to boost device outcoupling efficiency (see Figure 2).
We have fabricated antimonide-based PCSELs with a four-hole unit cell that deliver significantly improved efficiencies and somewhat smaller threshold currents. In comparison with sources with a photonic crystal having one air-pocket per unit cell and emitting about 10 mW of power, those with four pockets produce 30 mW, which is already adequate for many laser sensor applications.
Figure 3. Top view of the four-void unit cell, schematic of the side
view of the PCSEL device and corresponding camera images of the far
field patterns measured at 150 mA and 500 mA.
At a current just above the laser threshold, our four-hole PCSELs have a far field pattern that indicates a divergence angle of the order of a degree. This is a promising result, implying coherent operation over the whole 200 mm-diameter emitting aperture. Unfortunately, as the injection current increases, a cross-like shape appears in the far field pattern, as the beam broadens along orthogonal X-directions (see Figure 3). There is also some broadening of the laser emission spectrum, indicating excitation of higher-order lateral modes.
Our body of work highlights the benefits that can be realised with an air-pocket retaining regrowth methodology for antimonide-based PCSELs. We plan to build on this success by optimising the buried photonic crystal parameters of our devices, so that they can deliver stable single-spatial-mode operation and an increased external efficiency. Another objective is to extend the room-temperature continuous-wave operation of our GaSb-based PCSELs to 3 µm and beyond.
The work on III-V-Sb PCSELs at Stony Brook University is supported by US Army Research Office.